Background
Pathophysiology stems from pressure-related changes in the affected muscle.
Exact mechanisms are not clearly understood, but some models have been postulated.
Local pressure phenomena and reperfusion injury account for the clinical issues.
Diagnosis has been inaccurate which has impeded the full understanding of pathophysiology.
Inciting Factors in Early Compartment Syndrome
Traditional teaching is that acute compartment syndrome (ACS) occurs when locally increased tissue pressure compromises local circulation and neuromuscular function [1–5]. Circulatory patency is what maintains normal tissue function in the affected tissues including importantly nerves and muscles. Functional abnormality results after initiation of factors leading to ACS. Several markers of ACS have been used or sought and are currently seen as a direct result of the pathophysiology changes not as initiating factors. Trauma to the area results in swelling, ischemia, inflammation, patchy oxygen metabolism deficiencies, and increasing pressure [5, 6]. The question of which of these pathological changes comes first in the ACS scenario is a little like the chicken or the egg debate in that it may not be as important as the actual diagnosis. Hargens et al. [7] found normal capillary pressure to be between 20 and 33 mm Hg. Pressures above this have been deemed sufficient to shut off flow and cause ischemia. Normal interstitial fluid pressure is around 10 mmHg, a value fairly close to the capillary pressure. Authors [8] initially observed that with progressively higher applied external pressures, the blood flow to that area ceased before the difference between mean arterial and applied pressure became zero. This was the basis of the critical closure theory. A significant transmural pressure is theorized to maintain arteriolar patency. Tension in the walls of arterioles is actively produced by smooth muscle contraction. If tissue pressure is elevated enough, transmural pressure is insufficient for the arterioles to actively close and blood flow is arrested [9]. Support for critical closure was further gained from the studies of Ashton [8] on the effect of limb temperature. It was demonstrated that the critical transmural pressure varied with limb temperature in that a greater transmural pressure was required to maintain blood flow when local cooling increased the tone of arteriolar wall smooth muscle. Would critical closure be sufficient for prolonged compartmental ischemia? Ischemia causes vasodilation, which may bring more fluid into the affected compartment. Undoubtedly, this theory could be consistent with early ACS but probably does not explain ACS propagation in borderline cases. Other authors [10, 11] have discussed increases in tissue pressure being responsible for reduction in the local arteriovenous gradient and thereby local blood flow. When the metabolic demands of the tissue are insufficiently met by reduced flow from increased pressure, a compartmental syndrome may result. This theory does not dictate a zero-flow scenario and is therefore more reasonable and is a better model of ACS.
All of the factors that change the metabolism of the traumatic zone combined with anatomic limitations in blood supply, muscle fascial covering, and altered physiology result in ACS. Without a doubt the pressure increase is what is best understood as a pathological event or marker by care providers. Increased tissue pressure that compromises local circulation has been demonstrated by many researchers. The method of ascertaining this has changed over the years but has consistently showed that abnormally high pressures are present early in ACS [1, 2, 4–6, 12–19]. The pressure changes the ability of the local circulation to deliver oxygen to the tissue. Monitoring muscle PO2 has shown the balance between tissue oxygen delivery and tissue oxygen consumption [3, 5, 15–17]. Each zone of the affected area may have a slightly different PO2 in the initial stages of ACS. With increased swelling and pressure, the whole compartment begins to show the effects. No critical pressure has been observed as a magic tipping point where ACS is definite, and in fact some studies have shown compromise at pressure of 20 mmHg – lower than the currently held trigger point for surgery [10]. The disease process is just a spectrum of pressure changes where there is a greater compromise of muscle PO2 at higher tissue pressures. There are several proposed mechanisms of pressure-induced circulatory compromise. These include a starling resistor model for flow cessation, irreversible damage to small vessels, clotting mechanisms, and others. None of these models have really been shown to be the sole mechanism although pressure changes cause most of what is seen physiologically in the early stages.
Most investigators believed that the physiological changes were all pressure related. Sheridan et al. [20] inflated a latex balloon in a muscle compartment of a rabbit. They were looking at the response of nerve and muscle to the added pressure. The PO2 declined with increasing pressure from an initial control value of about 10 mmHg to a low of 2.8 mmHg at a compartment pressure of 90 mmHg. The integrity of the peroneal nerve and muscles was tested by direct electrical stimulation. Higher pressures and longer periods of pressure application produced more frequent functional losses. In the end, the authors felt that the pressure alone was a sufficient explanation for all changes seen in ACS. Increased tissue pressure also directly compromises neuromuscular function. Rorabeck and Clark [6] and Hargens et al. [7] slowed nerve conduction velocity by the pressurized infusion of the anterior leg compartment of dogs. In general, increased tissue pressure as low as 20 mm Hg affects tissue flow, and tissue circulation is decreased as the applied pressure is raised.
Vollmar et al. were interested in the microvascular response to similar external pressure elevation seen in ACS [21]. They used a skinfold model that was not an exact substitute for a compartment but illustrated a potential physiological change in tissue flow. They studied the response of the different segments of the microcirculation in terms of vasomotor control (change of vessel diameter) and cessation of blood flow with progressive changes in external tissue pressure. They felt that the study disproved the critical closing theory but complied with the hypothesis of reduced arteriovenous pressure gradients as the cause of blood flow decrease in compartment syndrome. They found that there was an increased perfusion pressure gradient needed in order to restart blood flow in small vessels. It was seen as a confirmation of the existence of so-called yield stress in microvessels. The high susceptibility of capillaries to elevated external pressure indicated to the authors that there was a need for early fasciotomy to restore impaired circulation. Lack of effective circulation is the factor that perpetuates further physiological changes and propagates a full compartment syndrome. It is the tipping point of the syndrome. The amount of pressure the muscles can tolerate before deficits are produced is also altered by local blood flow changes with examples being limb elevation, arterial occlusion, hypotension, or hemorrhage [10]. Dilation in the arteriole system caused by injury, along with collapsing smaller vessels and increased permeability, leads to increased fluid extravasation and raised interstitial fluid pressure. As it increases, perfusion to tissue becomes decreased. Once perfusion reaches a low level, tissue hypoxemia results. The combination of hypoxia, increase in oxidant stress, and development of hypoglycemia in the compartmental tissue causes cell edema due to a shutdown of the ATPase channels that maintain cellular osmotic balance [22]. Early ACS microvascular dysfunction results in a decrease in capillary perfusion and an increase in cellular injury and was associated with a severe acute inflammatory component [23]. The loss of cell-membrane potential results in an influx of chloride ions, leading to cellular swelling and ongoing cellular necrosis. The increase in tissue swelling worsens the hypoxic state and creates an ongoing positive feedback. As the cascade of elevated pressure then compromises the microcirculation with decreased oxygen and nutrient delivery, tissue anoxia with eventual myonecrosis then proceeds. In fact, systemic changes have been reported [24] as remote changes in liver and kidney function.
Changing Tissue Tolerance with Increased Pressure
Ongoing pressure and the tissue response are difficult to quantify. Tissues will react in different ways depending on the metabolic demands of the tissue and the duration of the increased pressure. This brings into play the specific effect of increased tissue pressure on local blood flow in the tissues. Bone will react differently than muscle and nerve – the more commonly injured tissues. Nerve and muscle do have a potential for recovery and reconstruction following ischemic injury. Hypotension, hemorrhage, arterial blood flow cessation, and limb elevation all reduce the tolerance of limbs for increased pressure [10]. Hargens et al. [7] elevated tissue pressure by the infusion of autologous plasma. They found some slowing of nerve conduction with a pressure of 30–40 mm Hg for 8–14 hours, but these conditions did not completely arrest nerve conduction. Pressure of 50 mm Hg for 330 minutes did stop nerve conduction. Sheridan et al. [20] inflated a leg balloon in rabbits to investigate the response of nerve and muscle to direct stimulation. Applied pressure of 60 mm Hg for 6 hours produced consistent functional losses. A pressure of 100 mm Hg for 12 hours caused a loss of all nerve or muscle stimulation response. Rorabeck and Clarke [6] found that 40 mm Hg reduced peroneal nerve conduction velocity from 40 to 30 m/sec over 2.5 hours. A pressure of 80 mm Hg arrested peroneal nerve conduction after 4 hours. Certainly, there is a difference amongst subjects and species for tolerance of pressure before nerve conduction slows or stops. There are no studies in the literature on muscle function after pressure initiation. Some researchers have looked at muscle degeneration after ACS conditions. Hargens et al. [7] investigated the effects of increased pressure in their model system using technetium-99 m stannous pyrophosphate. They found that pressures exceeding 20 mm Hg produced in a canine model a significant uptake of the label when maintained for 8 hours. From this point, the amount of uptake increased dramatically as higher pressures were applied. Rorabeck [6] found that when a pressure of 40 mm Hg was applied in a canine model, there was an increased in venous creatinine phosphokinase activity. Similar findings were noted for lactic dehydrogenase. They could not quantify the amount of marker with the amount of pressure applied. This may indicate that there is no hard-critical pressure in every situation or person. If we look at the hypothesis of reduced arteriovenous pressure gradients as the cause of flow cessation in compartment syndrome, then it explains that lower arterial pressure will decrease the pressure tolerance of tissue [10]. Hypotension from halothane anesthetic used for surgery over a 5-hour period was studied. The results showed that the circulatory effect of 60 mm Hg of compartmental pressure was much more apparent in the hypotensive animals. Zweifach [25] also investigated the effects on the pressure tolerance of rabbit limbs after an acute systemic hemorrhage of 20% of blood volume. Applied pressure of 40 mm Hg led to significantly greater reductions in nitrogen washout, muscle oxygenation, and action potentials in the hemorrhage group. They had seen similar results in a canine model. Limb elevation can reduce local arterial pressure; however, elevation alone cannot lower the limb’s venous pressure below the level of the local tissue pressure – that in the compartment. Thus for any given tissue pressure elevation of a limb above the supine position, there is a reduction in the local arteriovenous gradient. This means that a lower pressure is paradoxically sufficient to cause damage in an elevated limb [10]. This result of the arteriovenous gradient effect is clinically relevant in that it suggests that limbs with compartments showing signs of inadequate blood flow should not be elevated. Elevation will lower local arterial pressure but will not affect local venous pressure. ACS will evolve quicker than in non-elevated limbs.
Tissue Reperfusion as a Late Inciting Factor for Compartment Syndrome
Necrosis of compartment contents due to low oxygen and nutrient levels does occur eventually with prolonged high pressures. However, another mechanism for ACS propagation does take place with incomplete arterial occlusion or returning perfusion after ischemia. Reperfusion injury is tissue damage caused when blood supply returns to the compartment contents after a period of ischemia [26]. The absence of oxygen and nutrients during ischemia creates an environment whereby restoration of blood flow results in inflammation and oxidative damage rather than complete restoration of normal function [23]. This may occur after reperfusion but must also occur in the period of time where the microenvironment is fluctuating between flow and no-flow conditions at the cellular level. Normal microvascular perfusion is made up of mostly continuously perfused capillaries. Elevated compartment pressure results in a shift of perfusion toward intermittently perfused and non-perfused capillaries [21, 23, 27, 28], leading to low flow ischemic muscle areas. The metabolic demands of the tissue cannot be met, resulting in the production of reactive oxygen species and other inflammatory intermediaries [23]. During ischemia, there is a gradual depletion of intracellular stores of energy. There is a buildup of products of low oxygen metabolism, particularly lactic acid, with accompanying hydrogen ion accumulation [29]. Eventual cellular death occurs in some areas of the compartment. Unlike a complete reperfusion cycle, the defined phases of compartment content injury cannot be clearly delineated in low-flow ischemia. The reperfusion injury would not only persist throughout the duration of the ACS, but would be further intensified by surgical treatment that allows restoration of normal blood flow into the capillary bed. Reperfusion may cause harmful effects by washing out necessary precursors for energy formation. Production of oxygen free radicals and calcium influx both occur with disruption of oxidative rephosphorylation at the mitochondria level [23, 30]. Upregulation of neutrophil receptors and endothelial leucocyte adhesion molecules lead to the sequestration of white blood cells in the muscle (with a prolonged inflammatory response) with prolongation of the reperfusion injury. Capillary endothelium is also damaged by prolonged ischemia with a resultant increase in capillary permeability. Returning perfusion results in extravasation through the damaged areas with an increase in compartment volume. Lawendy et al. [24] demonstrated a two-hit inflammatory model with ACS and fasciotomy representing two hits of the systemic physiology. ACS causes a significant initial rise in the level of TNF-α and is followed by a second peak in the systemic levels of TNF-α after fasciotomy. The second peak is felt to be due to cellular debris, proinflammatory mediators, and cytokines gaining access to the systemic circulation leading to a systemic inflammatory response. Several cytokines are significantly elevated after a few hours of ICP elevation – TNF-α, IL-1β, GRO/KC, MCP-1, MIP-1α, and IL-1 – almost all of which are inflammatory [31]. Continued seeping fluid from damaged capillaries and muscle will only propagate the cascade that results in complete compartment syndrome.
The combination of multiple factors culminates in ACS. Ongoing changes at the cellular level represent early pressure-induced reversible ACS. The vacillating flow-no-flow scenario at the muscle level either causes limited local cellular death and changes or progresses through to complete ACS and more apparent clinical changes. This is microenvironment reperfusion injury propagation. The model of arteriovenous gradient as an explanation for ACS may be close to the truth. Our treatment for ACS results in a more compete reperfusion injury particularly when diagnosis is late. In summary, although many reasons for ACS have been suggested, the main marker for pathophysiological changes remains pressure in the early stages of the syndrome. Continued physiological changes later in the disease can be tracked by other markers in combination with pressure.
Sequelae of high local pressures in a muscle compartment is the main problem.
Several mechanisms have been postulated with arteriovenous gradient model being most realistic to clinical scenario.
Pressure interacts with local tissue compromise and changes the resistance to injury.
Inflammatory mediators and metabolites potentiate the reperfusion injury.
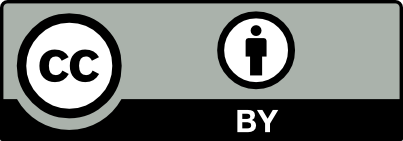
Open Access This chapter is licensed under the terms of the Creative Commons Attribution 4.0 International License (http://creativecommons.org/licenses/by/4.0/), which permits use, sharing, adaptation, distribution and reproduction in any medium or format, as long as you give appropriate credit to the original author(s) and the source, provide a link to the Creative Commons license and indicate if changes were made.
The images or other third party material in this chapter are included in the chapter's Creative Commons license, unless indicated otherwise in a credit line to the material. If material is not included in the chapter's Creative Commons license and your intended use is not permitted by statutory regulation or exceeds the permitted use, you will need to obtain permission directly from the copyright holder.