Time would flee, I subdue it.
In the early 1890s, after Étienne-Jules Marey’s successes with chronophotography ensured the continued viability of his “graphic method” of recording motion,
2 two German physiologists, Wilhelm Braune and Otto Fischer, set out to improve upon Marey’s techniques for the study of human locomotion. No mere dilettantes, Braune and Fischer were already well known in their field for studies of the human center of gravity and other investigations into the fundamentals of human motion. From 1889 to 1904 they published a series of studies of human locomotion, culminating in their landmark work,
Der Gang des Menschen (The Human Gait).
3 In their Leipzig laboratory, they dressed their experimental subject, a military recruit, in a black jersey and attached to him an elaborate mechanical scaffolding of incandescent tubes designed to illuminate his stride with short, intensely bright bursts of light (
fig. 1.1). They then photographed the subject as he walked, as comfortably as could be expected, across the darkened room. As he walked, the tubes fired, producing a strobe effect that was recorded through the camera’s open shutter. The resulting image—a series of white lines across a black background—became the basis for hundreds of calculations concerning the specific mechanics of human motion (
fig. 1.4). Interested in the economy of the laboring body—its most efficient conservation and expenditure of energy—they hoped their scientific analysis of human movement would lead eventually to a more efficient and productive society (or, at least, a more efficient soldier). Likewise, their improvements over Marey’s system of photographic measurement led to a much more analyzable and therefore “productive” chronophotographic image. After Braune and Fischer, photographs became even more efficient and productive tools of scientific research.
In Marburg in 1907, a young physicist named Max Seddig presented his dissertation on “Measurement of the Temperature Dependency of Brownian Motion.”
4 In an attempt to clarify the arguments for and against the atomic–kinetic model of heat, while also trying to provide experimental confirmation of Albert Einstein’s theories of Brownian motion, Seddig fashioned a device that could record chronophotographic images of microscopic particles affected by molecular activity. Seddig’s microscope–cinematograph combination supplied an objective record of Brownian motion from which he could calculate the velocity of these particles. Yet it was not the objective record that Seddig emphasized and praised but the machine’s ability to measure time intervals precisely. Alternatively, Heidelberg biologist Hermann Braus presented in 1911 the results of his application of a similar microscope–cinematograph combination to record and explore the growth of a tissue culture of a frog’s heart.
5 Using time-lapse cinematography, Braus sought to demonstrate that the culture actually grew rather than merely survived outside the organism. Unlike Seddig, he was not so concerned with measurement but with the temporal record of the event. With his motion picture record Braus was able to challenge competing claims about the growth of nerve cells.
These three cases—from human motion studies, physics, and biology, respectively—represent a fair sampling of the scientific use of film for research purposes in Germany before 1914. From these case studies we can draw some preliminary conclusions about the practical and philosophical connections between film and science. We might also be tempted, of course, to make general theoretical claims about the nature of cinema’s relationship to modern science and temporality.
6 But if we are to understand film’s role in modern scientific inquiry, we must temper expansive claims with more historically localized analyses of how film technology was actually
used.
7 What “film” meant to any given scientist depended very little on a theoretical conception of cinema in general; instead it depended primarily on how some specific incarnations of motion picture technology could be applied to the specific and historically contingent problems the scientist faced in his or her discipline. Hence any study of scientific research films must incorporate methods common to both the history of science and film history by investigating the manner in which research questions and media technology mutually influenced each other. What questions does a given discipline privilege at a given time? How do those questions shape—indeed, how are they shaped by—experimental design and available instruments? The appropriation of motion picture technology as a scientific research tool, its specific use within the laboratory, reveals the researcher’s assumptions about that which the camera is designed to capture. And these assumptions vary as widely as the different uses of film. But rather than making broad claims about what “science” or “cinema” is, thereby concealing this variety under top-down theoretical categories, we should let individual experiments reveal their assumptions and make our generalizations from those, if necessary. Design and deployment are themselves implicit theoretical statements.
If the cinematograph were an especially flexible tool that could be adapted to a number of different needs, these needs existed in the first place because of changes in the sciences themselves at the turn of the century. Biologists interested in cell development, for example, searched for new modes of visualization that would allow researchers a clear view of movement in time to resolve some heated disputes about the nature of cell growth; the techniques of tissue culture, on one hand, and those of motion pictures, on the other, offered two kinds of solutions, as we shall see. Physicists, too, looked to cinematography as a tool for investigating previously invisible phenomena, such as the effects of molecular movement, a topic of debates about the behavior of atomic phenomena. Seddig’s use of chronophotography and motion pictures reflects a common application of this technology in scientific research; while scientists admired cinematography’s ability to capture fleeting phenomena, they prized most highly film’s ability to decompose the event into discrete, regular units, which permitted measurement of its temporal and spatial components. Indeed, this particular use of motion picture technology, especially in the physical sciences, betrays an assumption about the event or phenomenon under study as itself discrete, divisible, determined by classical laws, regular, and—just like the filmstrip—reversible. Seddig’s use of motion pictures therefore demonstrates at once the value of film for scientific research and his (and Einstein’s) commitment to a particular understanding of the relationships between movement, time, and space—an understanding that French philosopher Henri Bergson was criticizing at precisely this moment in
Creative Evolution, his 1907 landmark study of the philosophy of biology. In short, as scientific disciplines reconceptualized the nature of matter, time, space, and the organism, they seized upon tools that could visualize these phenomena in accordance with these new concepts.
But film has never been just a convenient device, patiently waiting on the shelf as the scientist thinks up a new use for it as a solution to a new problem. Its availability and its existence also generated questions. Investigators’ interest in temporal phenomena was in part spurred by the arrival of a machine that could make the phenomena amenable for analysis. Furthermore, those scientific research programs that featured film technology were not only shaped by that technology, but their scientific method itself had certain features in common with the filmic apparatus. Scientific experiment shares with motion pictures an impulse to record immediately and directly, a willingness to manipulate time, and an inclination to isolate and quantify phenomena.
8 There are good practical reasons for using motion picture technology, of course. But in the late nineteenth century there developed also a
philosophical affinity between science and film that went beyond mere convenience. Cinema and science have come to share a certain way of thinking, so the history of the application of motion pictures in science can offer us a valuable opportunity to explore the relationship between science and modernity. Bergson was the first to suggest that science, through its parsing of continuous movement into discontinuous moments, proceeds in a way analogous to cinematography. If we are to understand fully the implications of the appropriation of motion picture technology by the scientific community, we must maintain a balance between the theoretical and the practical by considering this philosophical affinity alongside the way investigators put film to work in the laboratory. Bergson’s conception of science as inherently “cinematic” offers us a logical point of departure for such considerations. True enough, Bergson was not as popular in Germany as he was in France and the United States. But because I am interested in his thoughts on cinema and science in general—and not in his thoughts (if any) on particular applications in Germany or elsewhere—his historical impact on German culture is actually not relevant to my approach. Bergson’s theoretical framework can help us answer the question “What did cinema and science see in each other at this particular moment?” The individual case studies can reveal
how film was used; reading Bergson alongside these cases can help to reveal
why film was used. This chapter, then, will survey the use of motion pictures in the three case studies mentioned above.
Let me first reiterate the role of this chapter in the book’s overall goals. As I mentioned in the introduction, the larger argument (the “general theory,” if I may) concerns the correspondence and mutual accommodation between the logic of a discipline—its problem-solving patterns, its investigatory methods, its ideological assumptions—and film’s formal characteristics. This implies not just taking advantage of a medium-specific formal feature, such as temporal malleability (for example, time-lapse cinematography), to solve a representational problem, it also implies a functional or productive homology between this formal feature (for example, the linear regularity of time-lapse recording as a statistical sample of a larger unit of time) and a way of solving problems (the primacy of mathematics, for example) or of viewing the world (as naturally divisible into equal, regular units, for example). This match—between the formal features of the representational technology and the investigatory presumptions—matters, because it provides the researcher, community, or discipline with the reassuring sense that the tool will fit the task to which it is assigned. However, we must note that the match is ideally never perfect; otherwise there would be no new information. Experimental systems are designed to generate new questions, so there should be a dislocation or displacement between the more or less ideological assurance of this tool’s “worldview,” so to speak, and the strangeness of the view it provides.
9 Time-lapse cinematography can, for example, confirm an understanding of nature as regular and divisible, but it also offers a surprising, even thrilling new image that prompts new questions. The larger argument of this book is that the acceptance of any new (media) technology depends in part on this correspondence between some set of its formal features and the logic of the discipline. The specific argument (the “special theory”) is that expert vision expresses this disciplinary logic especially well and that film’s legitimacy within disciplines depended on its accommodation to expert modes of viewing.
But expert viewing is not the only way that disciplinary logic is expressed; the experimental system itself is also a manifestation of the discipline’s problem-solving patterns and theoretical presumptions. As Gaston Bachelard and others have argued, instruments are “theories materialized”: the design and deployment of experimental technology carries a preconception or preunderstanding of the phenomenon it is designed to isolate.
10 If we extend this system to include chronophotography or motion picture technology, we can see how the work of accommodating their formal features already made a statement about the relationship between the system and the object of study. Indeed, the work of creating a legible image—that is, understandable and acceptable to the discipline—reveals this relationship between system and object quite clearly. Likewise, the theory guiding the experimental observations is an expression of disciplinary logic. If instruments are theories materialized, then, as Hans-Jörg Rheinberger has noted, theories are also “machines idealized.”
11 Einstein’s theory of Brownian motion, for example, made several “shortcuts”—including the presumption of molecular two-dimensionality and velocity without direction, as we shall see—to manage the object mathematically and accommodate the phenomenon to both experimental confirmation and a particular disciplinary understanding of nature. Einstein’s mathematics, in other words, simultaneously became an “instrument” to guide observation
and a theoretical rendering of the experimental situation. To offer one more example, disciplinary logic can be expressed through the experimental system’s representational options. Film is only one part of an experimental system, of course, and only one in a range of representational tools that includes writings, sketches, graphs, and photographs. Selecting film as part of this media ensemble already implied a certain set of questions or needs. Hermann Braus, for example, found that using film was an especially powerful means of engendering belief among colleagues, while at the same time expressing, through its formal features (such as the duration of the projected image and its temporally forward motion) theoretical presumptions about cell growth. In this case, film and the new technology of tissue culture—also a representational tool—combined to express a new direction in the discipline’s visual needs and strategies.
So the correspondence and accommodation between experimental system or discipline and technology can happen in several ways, depending on the researcher’s or discipline’s goals and needs, which are locally and historically determined. This chapter will explore the “general theory” rather than the “special theory” of media technology’s disciplinary legitimacy. Specifically, this chapter will focus on three ways in which experimental systems incorporated chronophotographic or motion picture technology, especially on the correspondence and mutual accommodation between a given set of formal characteristics (of photography and/or film) and (1) an
object of study, (2) a
theory, and (3) the
representational options of an experimental system and discipline. Or, to put it another way, each of these three adaptations required a certain kind and amount of work to make the chronophotographic or filmic image into evidence. A close analysis of Braune and Fischer’s method will show how they created evidence in relation to their object of study (in this case, the human body). The discussion of Seddig’s experiment stresses the creation of evidence in relation to a theory (Einstein’s theory of Brownian motion). And the section on Braus emphasizes the creation of evidence in relation to a set of representational options within a discipline (here, cell biology). Each example deals to some extent with all three adaptations, of course, because they are intertwined, but the emphasis changes. In general, I will demonstrate that while the scientific community readily accepted chronophotography and film as valid instruments, investigators still had to perform considerable labor to adapt these devices to their tasks and to transform the resulting images into acceptable scientific evidence. Motion pictures may have allowed scientists to manage time and movement, but researchers first needed to manage film’s temporal rush and excessive detail. The nature of this work was shaped by its historical context. The subject of this chapter is therefore the way that investigators were continuously obliged to adapt as they juggled chronophotographic or motion picture technology, the needs of the individual experiment, the theories shaping the experiment, and the discipline’s priorities shaping the representational choices.
While observation is not an explicit focus of this chapter, it is inescapable. Braune and Fischer decomposed movement to train expert vision to phenomena that it might not see or have been able to see. Einstein’s theory of Brownian motion focused experimenters’ attention, showing them what to look for. For Braus, film was an observational tool that forced researchers to abandon previous theories and modes of analysis (which emphasized discontinuity) for an approach that emphasized synthesis and continuity. These case studies demonstrate that, as Ian Hacking has noted, experiment and observation are only heuristically separable.
12 Nevertheless, this chapter emphasizes other ways, besides observation, that disciplines accommodated the formal features of film and chronophotography. Expert vision is the explicit focus of the following chapters.
The chapter has five sections. It starts with a general overview of scientific research films (as opposed to popular science films) before World War I, in which I outline various prominent applications as well as some hypotheses about how motion pictures fit with the rhetorical goals of scientific enterprise. (A majority of scientific applications of film during the early period were devoted to various medical fields, which I will explore separately in
chapter 2.) The next section continues to explore why motion picture technology was intriguing to researchers; it focuses on Bergson’s discussion in
Creative Evolution of the relationship between cinema and science. In the third section, I place Braune and Fischer’s work on human locomotion in the context of both social modernity and the science of work. This section contains a detailed explication of Braune and Fischer’s method to show exactly how the merging of apparatus, image, and object actually occurs. The fourth section relates Seddig’s work to the general problems of atomistic physics at the turn of the century. In the fifth section, I sketch Braus’s cinematic contribution within the context of fin de siècle changes in the discipline of cell biology. The concluding paragraphs of this chapter compare the different cases directly to emphasize the mutual dependence between motion picture technology and emerging scientific agendas.
Scientists from a wide variety of disciplines—including but not limited to botany, military engineering, meteorology, neurology, psychology, and medicine, as well as the three already mentioned—were among the earliest users of motion picture technology.
13 Most histories of research films start with Jules Janssen, Eadweard Muybridge, and Étienne-Jules Marey, who were important transitional figures between scientific photography and motion pictures.
14 The initial adoption of moving images was relatively smooth because so much work had already gone into creating scientifically valid photographic images during the middle decades of the nineteenth century. The slow process by which still photography had been standardized—setting norms for emulsions, exposure times, preparation techniques, image interpretation, and so on—meant that the enthusiasm for photography often collided with rapidly evolving disciplinary requirements for scientific documentation. As Jennifer Tucker and others have shown, photography was not immediately and unconditionally accepted as a completely objective and scientific record.
15 Photography’s evidentiary status depended on its ability to meet several criteria of production and reception. Photographs had to withstand cross-examination by experts from any discipline to which they wished to testify; they were as subject to expert judgment as drawings or other illustrations.
16 In Germany, for example, microphotographs were not generally accepted as proper evidence by the scientific community until respected bacteriologist Robert Koch’s innovations and rhetorical efforts made “reading” photographs of bacteria a truly collective activity among an international group of scientists and physicians.
17 Yet by the 1890s, the protocols for generating acceptable scientific photographs had been established in most disciplines, so the innovation of motion pictures was greeted enthusiastically, their way smoothed by Marey’s renowned chronophotographs and graphic inscription methods.
18
Marey is indeed the most important figure in any history of early scientific film, because his efforts and resources shaped the application of motion pictures to scientific experiment. Marey was intrigued by Muybridge’s serial photography when he first encountered it in 1881 but ultimately disappointed in its scientific value: Muybridge’s 24-camera, trip-wire method of recording was prone to mechanical inaccuracy and incapable of managing the exact time intervals required for careful research. So at the Collège de France in the 1880s and 1890s Marey explored photographic and chronophotographic methods for visualizations of movement that accounted for distances and times more precisely. The Institut Marey was founded in 1901 to carry on his work; researchers such as Lucien Bull, Pierre Noguès, and Joachim-Léon Carvallo continued to explore the relationship between experiment and visualization, especially in the areas of slow and high-speed cinematography and X-ray cinematography. Marey’s assistant at the Collège de France, Charles Émile François-Franck, continued his work on microcinematography in particular; collaborating with Lucienne Chevroton, Fred Vlès, and others, François-Franck published widely on the chronophotographic and cinematographic recording of microscopic and macroscopic movement. These two sites were also magnets for individual researchers searching for novel ways to make visible their objects of study; French biologist Antoine Pizon and Swiss biologist Julius Ries both worked with the team at the Institut Marey to capture visually the process of cell division, for example, while François-Franck helped French physicist Victor Henri with his research into Brownian motion (I will have more to say about all of these examples later in the chapter).
Whether scientific cinema grew out of team efforts focusing on new visualization techniques or out of the work of individual researchers focused on experimental problems that motion pictures might solve, both models had one thing in common: the need for resources. Needless to say, the early motion picture apparatus was expensive, often cumbersome, and usually difficult to adapt. Its use in scientific circles, therefore, was generally restricted to established researchers who had the necessary financial and technical resources at their disposal. Indeed, the distribution of resources largely dictated the spread of motion picture technology in the laboratory. Thanks to Marey’s considerable political and scientific skill, France could boast at least two sites with the necessary budget and expertise to pursue such a program. Germany also had a university research infrastructure in place that allowed individual researchers to explore the use of motion picture technology, but no single site dominated.
19 Aside from the many medical applications, which we will examine closely in the next chapter, we can count botanist Wilhelm Pfeffer’s time-lapse chronophotography of plant growth at Leipzig University and Carl Cranz’s high-speed studies of ballistics at the military academy in Berlin-Charlottenburg as notable intersections of science and film at the university level.
20
Research film also received a boost from manufacturers who recognized that lending their equipment and funds provided not only a measure of legitimacy and good press but entertainment for movie-going audiences as well. Pathé Frérès, for example, funded the filmmaking of microcinematographer Jean Comandon and then distributed his films to theaters around the world.
21 Occasionally a German manufacturer would lend a hand to researchers; film pioneer Oskar Messter, for example, had pretensions in this arena, and companies such as Ernemann were sometimes acknowledged as technical patrons.
22 But even researchers who purchased the basic apparatus from a manufacturer such as Lumière or Ernemann were still obliged to make modifications to the equipment in their own laboratory setting. Carl Zeiss’s optical laboratory in Jena, for example, seems to have been especially suited to this kind of work. Generally speaking, despite the involvement of some film manufacturers, these films were usually made by specialists to be shown to other specialists at scientific conferences or in the lecture hall.
This is not to say, however, that scientific films were limited exclusively to an elite audience. Scientific titles were often part of the program at the local movie theater. Companies such as Pathé, Gaumont, and Éclair in France or Urban in England, especially, produced their own series of scientific films for general audiences.
23 German audiences would have been familiar with this genre from the titles imported by these companies. (Foreign manufacturers generally dominated the German exhibition market until the 1910s.) In addition, the German periodical
Film und Lichtbild (a German
Popular Science for film enthusiasts) acted as something of a clearinghouse and ad hoc distribution company by publishing lists of scientific films and offering discounts to its readers.
24 There were also dedicated screenings occasionally,
25 as well as theaters devoted to the genre, such as the Fata Morgana theater in Dresden, which opened in August 1912 and showed only scientific, industrial, and nonfiction films.
26
But what of the research films of a Seddig or a Braus? Did they ever find their way to the public? It is very difficult to say without a complete survey and correlation of all films made in the laboratory with those screened in public or semipublic venues—a task that is likely impossible to complete. There are good reasons for both possible answers, yes and no. On one hand, these films were the result of considerable expense and effort, so investigators might have been reluctant to give them up for duplication.
27 (Comandon and others like him were exceptions, because they made legitimate research films for hire.) Also, some scientists might have hesitated to cross the line between serious research and its popularization. Motion pictures already had acquired the yellow tinge of mass culture, so some investigators were probably reluctant to have their work completely jaundiced by a matinee showing at the local nickelodeon. Not that the scientific application of motion pictures encountered serious objection; while film histories often repeat legends of academic hostility to researchers using motion pictures in their experiments (usually limited to French medical films and the Doyen controversy, described in
chapter 2), evidence of such protests is actually rare in comparison with the general enthusiasm displayed for the new technology. On the other hand, research films were the topic of a considerable number of screenings and discussions, and teachers, reformers, and even scientists encouraged these ventures into the popular realm as important efforts at public outreach.
28 Furthermore, there were already a number of screenings of these films in the semipublic realm of the university lecture, so it would not have been too much of a leap to take the next step.
29 And as manufacturers such as Messter and Ernemann lent their equipment and expertise to researchers, they may have asked for copies of the films, which might have been made available for rental in the manufacturer’s catalog.
30 It seems that the public screening of any given laboratory film depended on the resources and predilections of the individual researcher or the manufacturer; I have not yet found a consistent conduit in Germany between the scientific laboratory and the movie theater.
By and large, then, these films served primarily as a form of scientific evidence. Despite its mass culture connotations, its high cost, the high level of technical expertise required, and the often futile results, motion picture technology offered several tempting benefits to the researcher. Like still photography, the motion picture camera provided a mechanical, automatic, hence “objective” record, thereby adding substantial evidentiary weight to scientific claims.
31 The photographic image, like other graphic inscription devices (such as the electrocardiograph), seemed to provide researchers with an unmediated and permanent record of any given phenomenon, one that could be stored and disseminated with ease. And because it could be projected and reproduced, the photographic image proved useful for demonstrations as well as experiments; indeed, a motion picture projector was just as likely to be found in a lecture hall as in a laboratory.
32
Unlike still photography, however, the motion picture had the capacity to record events as they occurred over time. This singular feature offered several advantages. The camera itself could act as a mechanical, indefatigable prosthesis of the scientist’s eye, a tireless observer of events that was able to catch the slightest change without the interruption of a blink. Furthermore, motion pictures offered the scientist the option of manipulating time by recording (or projecting) the film at different speeds. Slow-moving events could be sped up with time-lapse techniques; fast-moving phenomena could be slowed down through high-speed cinematography. As a result, temporal events invisible to our ordinary perception became “visible”; film became a kind of temporal microscope or telescope, bringing nature’s aloof wonders closer to our level. Finally, as implied above, the motion picture camera could also act as a precise measuring tool. By controlling the rate at which the film passed through the camera as the phenomenon traveled a set distance, the scientist could then calculate the speed of the recorded movement. This ability was by far the most intriguing aspect of cinema’s scientific potential, and researchers spent considerable energy perfecting it.
Motion picture technology, then, was an especially flexible tool that could be adapted to a number of different tasks. In this respect, however, it is no different than any number of technical innovations adapted for scientific use, from perspective drawing to the computer. Successful adaptation depends on a variety of circumstances, but as sociologist of science Bruno Latour has argued, the most salient predictor of which technologies the scientific community will adopt is the extent to which the adoption will aid the community in rhetorical struggles. Latour maintains that technologies that serve as inscription devices, or “writing and imaging procedures,” function rhetorically in debates between authors and groups as tools that help “in the mustering, the presentation, the increase, the effective alignment, or ensuring the fidelity of new allies.”
33 Struggles between scientists are the same as those between generals and politicians, Latour argues; those with the most allies win. Therefore, the essential characteristics of any inscription device—the qualities that will ensure its success in the scientific arena—have less to do with its ability to provide accurate inscription (visualization, writing) per se than whether those properties can be put to use in rhetorical struggles. Latour explains:
In other words, it is not
perception which is at stake in this problem of visualization and cognition. New inscriptions, and new ways of perceiving them, are the results of something deeper. If you wish to go out of
your way and come back heavily equipped so as to force others to go out of
their ways, the main problem to solve is that of
mobilization. You have to go and to come back
with the “things” if your moves are not to be wasted. But the “things” have to be able to withstand the return trip without withering away. Further requirements: the “things” you gathered and displaced have to be presentable all at once to those you want to convince and who did not go there. In sum, you have to invent objects which have the properties of being
mobile but also
immutable,
presentable,
readable, and
combinable with one another.
34
Motion picture technology had all of the qualities of this sort of immutable mobile, a good indicator of its success as a scientific instrument. The instrument itself was mobile, but more importantly, so were the films. “Immutability,” in Latour’s sense, refers to the permanency of both the inscription process and the object or condition represented. Simply, the inscription must be relatively permanent, as films were, while providing a translation without any seeming corruption of the thing represented. The photographic image’s necessary physical connection (via light and chemical processes) to the object represented served to guarantee that the object was relatively uncorrupted by the recording process. The films were meant to be projected, so they were of course presentable, but because they were also photographs, they could be presented in a wide variety of ways, as illustrations in journal articles or as lecture slides, for example. In this way, the films could also be combined with other technologies, such as print technology, but the apparatus itself could be combined with others as well, such as the microscope. The “readability” or legibility of the technology is the most contentious aspect of any innovation, because the interpretation of new forms of inscription always requires negotiation within a discipline. Experts and innovators haggle over the meaning of signs until standards of production and protocols of interpretation emerge.
35 Generally speaking, however, motion pictures, like photography before them, were considered very legible for scientific purposes.
Without a doubt, motion pictures presented scientists with new analytic techniques that could manipulate time and space. However, at the same time, science’s very mode of analysis was newly subject to debate. At least since the romantics, some German thinkers had come to associate science’s empirical, experimental, and materialist method with a cold, mechanistic, and “disenchanted” view of the world. As Anne Harrington has argued, the rebellion against mechanistic, reductionist science often consisted of calls for “wholeness” in various forms, from Hans Driesch’s biological vitalism to Gestalt psychology to Richard Wagner’s
Gesamtkunstwerk.
36 This reaction was not limited to Germany, of course; perhaps the most prominent figurehead of this rebellion was Henri Bergson, whose philosophy—or its popularization—spread like wildfire through the parlors and lecture halls of Europe and the United States in the years before World War I. If film was still a relatively marginal research technology at this time, Bergson’s
Creative Evolution brought it to the center of the debates about scientific method.
37
Creative Evolution, which appeared in 1907 and quickly became Bergson’s most famous work, was concerned with evolutionary biology in the same manner that his earlier
Matter and Memory (1896) was concerned with psychology and his later
Duration and Simultaneity (1922) dealt with physics. In
Creative Evolution, he took the evolution of life as a fact, but expressed dissatisfaction with scientific explanations of it. According to Bergson, the mechanistic approach to biology perpetuates a common analytic mistake: it divides up organisms and living processes in order to understand their parts, but does not, because of this division, fully understand their total, living reality.
38 Consequently, the analytic, mechanistic, reductionist approach cannot satisfactorily explain change or the creation of new forms or new solutions. Bergson was intent to bind the organism’s “living reality” to the flow of time. That is, Bergson made the flow of time, which we all experience as incessant and forward moving, the model of life itself. The central concept here is his notion of
durée, or “duration.” Bergson insisted that we make a category mistake when we divide the continuity of lived experience into the discontinuity of a series of separate points. Bergson reversed the traditional or commonsensical view that change is a succession of states, a view that makes those states logically anterior to change. Bergson’s view, instead, was that change is primary and that to analyze change by breaking it into a succession of states misrepresents the true reality of the world, which exists in constant flux, “becoming,” or
durée. Organisms exist in time, in
durée, and cannot be separated from it without losing an understanding of their lived reality.
This same logic applies to the examination of motion. Bergson saw the universe in a constant state of flux; change and movement are the only constants, the only true reality. Matter, form, or solidity are only stable views of this essential instability. Unfortunately, our common, ordinary, analytic perception cannot grasp this flux; it can only extract determinate moments, which we then mistake for an accurate picture of reality. Even our body is not solid but “is changing form at every moment; or rather, there is no form, since form is immobile and the reality is movement. What is real is the continual
change of form:
form is only a snapshot view of a transition…. [Therefore,] our perception manages to solidify into discontinuous images the fluid continuity of the real” (328, emphasis in original). It is not so much that this mode of perception is wrong, it is just that it is incomplete and should not be mistaken for a true understanding of the world. Bergson acknowledged that human beings cannot help but think this way, dividing the flow of the world and time into discrete intervals, but he insisted that this habit of thought should be overcome: “to invert the habitual direction of the work of thought.”
39 That is, given that analysis or this way of viewing the world is more or less habitual and necessary, Bergson declared that to recognize
durée, “The mind must do violence to itself, has to reverse the direction of the operation by which it habitually thinks, has perpetually to revise, or rather to recast, all its categories.”
40 Bergson’s philosophy, then, was an attempt to save us from our logical errors of thought so that we could see the world in a different way. Specifically, he argued against two category mistakes: mistaking discontinuity for continuity and space for time.
Bergson used the example of motion pictures, which consist of a series of still images projected to imitate real movement, to illustrate the relationship between the continuity of durée and the discontinuity of our ordinary, analytic perception. The mechanism of cinema was analogous to, even expressed, our ordinary perceptual process. This process, Bergson elaborated,
consists in extracting from all the movements peculiar to all the figures an impersonal movement abstract and simple,
movement in general, so to speak: we put this into the apparatus, and we reconstitute the individuality of each particular movement by combining this nameless movement with the personal attitudes. Such is the contrivance of the cinematograph. And such is also that of our knowledge…. Whether we would think becoming, or express it, or even perceive it, we hardly do anything else than set a kind of cinematograph inside us. We may therefore sum up what we have been saying in the conclusion that the
mechanism of our ordinary knowledge is of a cinematographical kind. (332, emphasis in original)
What does it mean to “set a kind of cinematograph inside us”? It means that when we try to think movement or change, we cannot help but to conceive it first as a series of individual states of being or “snapshots” of form. The accumulation of these “snapshots” provides us with a conception of movement, a conception as illusory as the movement of the cinematic image at twenty-four frames per second. Thinking of movement or change as a series of states—rather than as a thing in itself, as Bergson urged us to do—forces us to extrapolate movement from the series. With that extrapolation, movement becomes universal, ideal, or “movement in general.” We attempt to reconstitute the particularity of the movement by an effort of synthesis, which is what the accumulation of snapshots amounts to. Out of habit, our ordinary way of thinking is cinematic.
In the same way, modern science “proceeds according to the cinematographical method,” in that “it is the essence of science to handle signs, which it substitutes for the objects themselves” (357). These signs “denote a fixed aspect of the reality under an arrested form. To think movement, a constantly renewed effort of the mind is necessary. Signs are made to dispense us with this effort by substituting, for the moving continuity of things, an artificial reconstruction which is its equivalent in practice and has the advantage of being easily handled” (ibid.). According to Bergson, science is not really able to understand movement per se, movement as continual flux. Yes, it can understand it as the difference between the changes of two stable states, but it cannot grasp the essential dynamism of change. Instead, the scientific approach creates abstractions—the geometric understanding of movement as a line or the mathematical understanding of movement as an equation—to help us grasp that which is in constant motion. For Bergson, the normal scientific approach to movement proceeds by leaps from moment to moment, from arrangement to rearrangement; science may increase the number of moments it isolates “but it always isolates moments…. It does not bear on the interval, but only on extremities” (357–358). To the extent that science followed this tendency to divide movement into stable units and to treat the interval as an enabling ellipsis and not as precisely the problem, modern science for Bergson was essentially cinematic.
Bergson characterized the history of science in these terms as well. Aristotelian science was also cinematic, but it differed from modern science in the way it broke up time. For the ancients, the time of the movement of a falling body, for example, had certain determinate periods, whose natural articulations, like puberty, presented moments when there occurred the natural release of a new form. For modern science, according to Bergson, time “has no natural articulations. We can, we ought to, divide it as we please. All moments count. None of them has the right to set itself up as a moment that represents or dominates the others. And, consequently, we know a change only when we are able to determine what it is about at any one of its moments” (360). Time, then, has become democratic in the modern age; there are no more privileged moments. This democratization marks the difference between the
qualitative description of the ancients and the
quantitative measurement of the moderns (361). Modern science works only with a view to measure, and it selects as its objects only those phenomena that can be measured. “It retains only the events or systems of events that can be thus isolated without being made to undergo too profound a deformation, because only these lend themselves to the application of its method. Our physics dates from the day when it was known how to isolate such systems” (371–372). In the same way, motion picture technology divides temporal events evenly, into a neat twenty-four frames per second, for example. The precise and democratic division of time common to the cinematic apparatus mimics modern science’s insistence that no moment be privileged over another.
In this way, Bergson outlined the deep affinity between cinema and science, one that at least partially explains their immediate mutual attraction. But if Bergson condemned our “ordinary perception” and therefore cinema (and scientific method) as incomplete—thereby joining the rebellion against mechanistic science—he nevertheless recognized that cinema had potential beyond its analytic character.
41 In an interview from 1914, Bergson regarded motion pictures somewhat more sympathetically:
Several years ago, I went to the cinema. I saw it at its origins. Obviously, this invention, a complement to instant photography, can suggest new ideas to a philosopher. It could be an aid to the synthesis of memory, or even of thought. If the circumference [of a circle] is composed of a series of points, memory is, like cinema, a series of images. Immobile, it is in a neutral state; in movement, it is life itself.
42
Bergson recognized that cinema had the capacity to provide both analysis and synthesis, that it had an inherently ambiguous character precisely because it is both still and moving. Indeed, Bergson’s invocation of the cinematographic apparatus was never an outright condemnation of the cinema but instead a way of describing a tendency in our habitual, everyday way of thinking that science had codified into experimental method.
43 If the point of his philosophical project was to “reverse” this way of thinking toward a truer, less alienating view of the world—toward an embrace of “intuition”—then there was also the possibility that cinema could somehow participate in that reversal. In other words, if modernity—“the alienating, blinding experience of the age of large-scale industrialism” to which, Walter Benjamin claims, Bergson’s work responded
44—exacerbated this alienating perception, cinema’s analytic character aligned it with that alienation, while its synthetic nature associated it with authentic experience. Cinema was both a social irritant and amelioration. In other words, the Bergsonian tension between continuity and discontinuity, between unity and fragmentation, was an expression of “the alienating, blinding experience of the age of large-scale industrialism,” but it was also a tension expressed in the very form of film itself. The aspect of cinema that made it a significant tool for scientific analysis existed alongside the aspect of cinema that made it useful for philosophical thinking.
While Bergson’s philosophy struck a chord throughout Europe and the United States, its resonance was muted in Germany, where the reception of his work was not quite as overwhelming.
45 Nevertheless, many German thinkers struck notes in much the same key. Wilhelm Dilthey, for example, argued that positivist philosophy and science alone could not grasp the whole of life’s rich variety: “The basic conception of my philosophy is that up to now no one has put whole, full, and unmutilated experience at the basis of philosophizing, that is to say, the whole and full reality.”
46 According to Dilthey, this “whole and full reality” could not be fully understood using the “mutilating” knives of reductionist analysis and causal explanation.
47 So he proposed his famous division between the physical sciences, which rely on causal explanation, and the human sciences, which derive their insights from empathetic or hermeneutic understanding: “The basis of the human studies is not conceptualization but total awareness of a mental state and its reconstruction based on empathy.”
48 This recalls Bergson’s conclusion about the incompleteness of the scientific enterprise:
It seems then that, parallel to this physics, a second kind of knowledge ought to have grown up, which could have retained what physics allowed to escape. On the flux itself of duration, science neither would nor could lay hold, bound as it was to the cinematographical method. This second kind of knowledge would have set the cinematographical method aside. It would have called upon the mind to renounce its most cherished habits. It is within becoming that it would have transported us by an effort of sympathy. (372)
In other words, both philosophers were interested in opposing the machine of scientific method with the wholeness offered by a certain intuitive, empathetic understanding. Cinema, via Bergson, stood squarely in the middle of these debates about scientific method in fin de siècle Germany. Its inherently ambiguous character was reflected in the different ways scientists used it in their experiments and in the different ends to which it was employed. It is true that Bergson in Creative Evolution emphasized only the analytic potential of motion picture frames—that is, the use of celluloid as a “mutilating” dissection knife trained on living movement. Yet scientists in their actual use of film also effectively employed the projected film as an end in itself, revealing what Bergson would later recognize (and Gilles Deleuze would still later describe) as cinema’s and science’s capacities for synthesis within a persuasive image of movement itself. In the rest of this chapter, I will outline some of these different means and ends by examining a series of case studies of the appropriation of cinematic technology in the science of work, in physics, and in cell biology.
To illustrate his point that “with immobility set beside immobility, even endlessly, we could never make movement,” Bergson suggested this thought experiment:
Suppose we wish to portray on a screen a living picture, such as the marching past of a regiment. There is one way in which it might first occur to us to do it. That would be to cut out jointed figures representing the soldiers, to give each of them the movement of marching, a movement varying from individual to individual although common to the human species, and to throw the whole on the screen. We should need to spend on this little game an enormous amount of work, and even then we should obtain but a very poor result: how could it, at its best, reproduce the suppleness and variety of life? (331)
This “little game” bears a remarkable similarity to the serious experiments of Braune and Fischer, who used the marches of a military recruit to extract principles of motion “common to the human species” from his “individual movement.” Their results, after “an enormous amount of work,” look very much like “jointed figures,” although Braune and Fischer would protest that their representations were not meant to convey “the suppleness and variety of life” but rather the universal laws that subtend it. Interested primarily in isolating moments in motion and reducing them to universal principles, they were unapologetic contributors to the mechanistic, positivist, analytic trend in the sciences. In this section, I will use their work as an example of the “cinematographical method” in science that Bergson interrogates. But I also want to demonstrate how their agenda fits into larger social concerns about modernity. So I will first survey the science of work and its promise to offer a way to manage social problems and class relations. The military heritage of this discipline leads to a consideration of the “docile body” in the science of work and scientific photography or cinematography. Then I will turn to the actual process by which scientific images are rendered, by reading their technique as exemplary of the transformation of the chronophotographic image into acceptable evidence.
Before the image could be accepted as evidence, the subject itself had to be made manageable. At each stage of Braune and Fischer’s experiment, they had to make adjustments to the subject, the apparatus, and the means of analysis. The recruit’s body, for example, had to be continually manipulated and adjusted to create a legible image. Indeed, just as adapting the subject’s body to the apparatus, and vice versa, played a large role in creating and legitimating a scientific image, so adapting labor to the machine age was the primary goal of the science of work. Likewise, Braune and Fischer’s intricate efforts to prepare the military recruit for his mission correspond nicely with the mathematical contortions required to translate the image into acceptable scientific data. Understanding the mechanics of the human body required its submission and disassembly on both sides of the equation, before and after the chronophotographic inscription. As we shall see, disassembly, or analysis, was the first step to rebuilding the body and society. Braune and Fischer used the apparatus (both the camera and its accompanying scholarly translations) to break down human movement into its constituent parts. But the painstaking work of analyzing each movement would be rewarded only if they could find a way to eliminate needless effort and bring all the elements back together again so that they worked more efficiently, all in the name of conserving energy and forestalling fatigue.
As Anson Rabinbach has persuasively demonstrated, the twin concepts of “energy” and “fatigue” were enormously powerful tropes for scientists and reformers of fin de siècle Europe.
49 New scientific models of energy consumption and conservation, along with the ubiquitous technologies and techniques of mass production that accompanied the second industrial revolution, led many scientists to think of the human body as a motor, a machine governed by the same laws of physics and chemistry as its man-made counterparts. The centuries-old battle between vitalism and mechanism heated up once again as this new mechanistic theory of the human motor encountered the critical vitalism, or
Lebensphilosophie, of Bergson, Driesch, and others.
50 As the demands of adapting human labor to new industrial techniques grew, a new science—the science of work—adopted these mechanistic principles. The science of work studied the “human motor” in order to define its laws and track down the origin of fatigue.
Yet the trope of fatigue was more than a scientific mania of the age; it expressed a profound concern over decline and social disintegration. As the structural changes wrought by the Industrial Revolution rippled through society, there arose a tendency “to locate the body as the site where social deformations and dislocations can be most easily observed” (21). Metaphors of health and sickness were used to express national anxiety. Fatigue became more than a physical ailment, it became a moral problem, a sign of weakness and absence of will. According to Rabinbach, “In fatigue the physical horizon of the body’s forces was identified with the moral horizon of the species; the moral infirmity of the population was directly proportional to the debilitating effects of fatigue…. [Furthermore,] fatigue represented the membrane between morally sacrosanct labor and the violent, irrational impulses that constantly threatened to disrupt social order” (43). The tensions between human labor, capital, and the machine age demanded some sort of solution, and European scientists studying the nature of work believed that greater productivity was the key to social harmony. Investigations into the nature of fatigue were hopeful steps toward resolving class conflict scientifically, that is to say, “neutrally.”
The science of work, which emerged in the nineteenth century on the periphery of scientific study, compensated for this marginal status by becoming a self-consciously international phenomenon. The relatively small group of researchers interested in this topic around the 1880s had very little support, institutional or otherwise. Ernest Solvay, a Belgian chemist, who conceived of society as “an enormous industrial enterprise dedicated to increasing overall productivity while encouraging social justice,” endowed the Institut de Sociologie in Brussels as part of his plan to study the nature of energy and fatigue.
51 Angelo Mosso of Turin, an Italian physiologist and educational reformer, invented the first efficient and accurate measure of fatigue, the ergograph, in 1884.
52 But it was Hermann von Helmholtz of Germany and Étienne-Jules Marey of France, in particular, who gave direction and means to the fledgling science.
Helmholtz’s contributions to science are legion, but he is perhaps best known for his version of the first law of thermodynamics, or the law of conservation of energy, which holds that energy is neither created nor destroyed but simply transferred.
53 His mathematical formulation of this fundamental law of physics provided science with its most substantive version yet, and it soon became more than a truism. This law’s companion axiom, the second law of thermodynamics, which was formulated by other scientists, including Rudolf Clausius and William Thomson (Lord Kelvin), also gained credence beyond the world of physics. The second law explained the process of
entropy, which holds that although energy cannot be destroyed, it tends over time to be degraded from useful forms to uselessness. The best example of entropy is the phenomenon of heat transference: when a hot body is placed next to a cold one, the potentially useful energy of the former will transfer to the latter until both have equal, and less useful, temperatures. The relevance of these laws of conservation and entropy was soon extended from the study of molecules to that of the human body and even to society as a whole. Fatigue became the corporal analog of the second law of thermodynamics, and degeneration became its social equivalent (45–48). Scientists, reformers, and opinion makers of all sorts soon began to depict the nature and problems of society in terms of energy, attention, will, and utility, on one hand, and fatigue, degeneration, entropy, and uselessness, on the other.
54 The laws of thermodynamics therefore provided the metaphors that motivated social plans for managing the conflicts and excesses of industrial society.
On the other hand, Marey provided the means by which scientists could study the laws of energy and entropy as they were played out through the human body. Marey, a physiologist who made lasting contributions to the fields of cardiology, physiological instrumentation, and aviation, as well as to the craft of photography and the science of work, provided not only the means for a minute analysis of the movements of the human body but the basis upon which these studies could be counted as legible and legitimate areas of inquiry.
55 His transformation of ephemeral phenomena, such as movement, into scientifically acceptable (that is, legible by disciplinary standards) visual evidence through his “graphic method” prompted a flood of motion studies that overran journals in the 1890s. In this respect, Marey is an exemplary figure in late nineteenth-century European positivism. His graphic method signaled the ascendancy of the process-oriented approach in physiology that has dominated the field for the past 150 years. His focus on the disassembly and reformation (in the broadest possible sense) of the human body is representative of the general goal of positivistic, inductive, physical sciences: analyzing individual instances of natural phenomena and provisionally concluding from them an ideal model or set of laws. As Rabinbach demonstrates, Marey represents the forging of a crucial link between cultural and social modernity, between late nineteenth-century disruptions in the perception of time and space and the efforts to manage the contemporary social crises (84–88). His kymograph and chronophotographs codified, even embodied, the historical confluence of these forces, giving the science of work direction, means, and legitimacy.
Designed to calculate “the mechanical work expended in different movements,”
56 Marey’s chronophotographs were also a link between cultural and social modernity, providing the basis for both a new leisure activity and a science of labor. Marey intended his “ergonomics”—or science of efficient movement—to lead to greater productivity. The concept of “training” was very important for Marey’s ergonomics and the science of work in general. Marey maintained that all animal locomotion is characterized by the transformation of abrupt and disjointed movements into consistent motion. (This is also an accurate description of the technological principle of cinema.) The central feature of all work—whether of humans or machines—is the transformation of irregular, inconsistent, and jarring shocks into regular and uniform activity. The body’s own elasticity permits the suppression of shock into regular effort. Muscles, for example, act to turn abrupt movements into dynamic work. Marey believed that animal and human motors are naturally efficient yet capable of improvement. His chronophotography attempted to demonstrate the potential for greater economy to be attained from “training”—essentially a program of scientific and bodily discipline. Through careful study of the movement of the body in various stages of a work process—whether forging iron, for example, or pole-vaulting—scientists could spot and correct inefficient movements, thereby showing the worker how he or she might expend the least amount of force and consequently accomplish the task with the least amount of fatigue.
57
Braune and Fischer’s studies of human motion extended this tradition.
58 To “investigate the influence of a relatively heavy load on gait,” they asked their experimental subject to carry “an army regulation knapsack, three full cartridge pouches and an 88 rifle in the ‘shoulder-arms’ position” in a number of different experimental settings.
59 These studies, ostensibly dealing “only with the experimental determination of the process of movement, without considering the cause,” were designed to recreate the process of labored movement in the hopes that these activities could be improved ergonomically.
60 In this they shared the approach and goal of other practitioners of the physiological branch of the German science of work, or
Arbeitswissenschaft, such as Nathan Zuntz and Wilhelm Schumberg, whose
Studien zu einer Physiologie des Marsches “surveyed all aspects of military drill” in the hopes of pinpointing the causes and consequences of fatigue and march-related illnesses.
61 The military orientation was a common motif in the science of work: Braune and Fischer’s experiments were supported by the German High Ministry; Marey stressed the potential benefits of motion study for military training in his numerous appeals for support from the French government;
62 Wilhelm Weichardt tested his infamous “fatigue vaccine” on the Austro-Hungarian army;
63 and Mosso tested his ideas about the relationship of mental and physical fatigue on Italian soldiers.
64
It is not too surprising that European theories of efficiency, elasticity, and fatigue often took an explicitly military orientation, considering that a military motto might be “efficiency through training.” As a matter of fact, the science of work was the industrial application of forms of discipline first deployed in the military. The temporal decomposition and reconstitution of the human body through chronophotographic or cinematic means also fit well into such agendas.
Moving Picture World once noted, “The United States Army has had [motion] pictures taken of a soldier going through the manual of arms. Thumb books with these pictures are made up and furnished to the recruit, who by looking carefully through them can easily trace every minute movement that goes to make up the completed action.”
65 In this example the recruit was expected to incorporate the lessons of the cinematic image in much the same way that he was expected to embody military ideology. The point is not so much that cinema and the science of work cooperated with the military but that the military application of motion pictures and of these scientific principles point to a coincidence of techniques that provide further insight into the relationship between science and cinema. Michel Foucault cast some light onto the larger intellectual history connecting the science of work and the state:
The great book of Man-the-Machine was written simultaneously on two registers: the anatomico-metaphysical register, of which Descartes wrote the first pages and which the physicians and philosophers continued, and the technico-political register, which was constituted by a whole set of regulations and by empirical and calculated methods relating to the army, the school and the hospital, for controlling and correcting the operations of the body. These two registers are quite distinct, since it was a question, on one hand, of submission and use, and on the other, of functioning and explanation: there was a useful body and an intelligible body.
66
But these registers, Foucault continues, overlap in the notion of “‘docility,’ which joins the analyzable body to the manipulable body. A body is docile that may be subjected, used, transformed and improved.” The scientist’s efforts to survey the human body and the colonel’s attempts to modify it both required that the body submit to a regimen of exercises. This may have included a series of measurements, tests, recordings, or it might have meant calisthenics in the morning and full-load drills in the afternoon. Either way, whether under the rubric of science or the state, docility, according to Foucault, “implies an uninterrupted, constant coercion, supervising the processes of the activity rather than its result and it is exercised according to a codification that partitions as closely as possible time, space, movement.”
67
This last sentence is certainly an apt description of Braune and Fischer’s experimental procedure, especially in that their chronophotographs “partition as closely as possible time, space, movement.” Foucault called these methods “disciplines,” and we would include science among them, because its work is essentially that of domestication. We may, after Ian Hacking, divide the work of the scientist into two types: representing and intervening.
68 Hacking equated this division, generally speaking, with the split between theory and experiment, even while acknowledging that the two are inseparable. Indeed, experiment is tightly bound with the process of representing, as sociologists of science have shown. But whether through representation or experimentation, any phenomenon to be studied must be “tamed” before it can become scientific data. In the attempt to analyze (or, more accurately, render analyzable) natural phenomena, the work of the scientist involves any number of phases, such as selecting, partitioning, measuring, or representing. It is impossible to present a phenomenon in its “natural” state; it must be rendered into material images, such as graphs, photographs, tables, charts, and diagrams—representations that function as Bergson’s “snapshot” of duration. Sociologist of science Michael Lynch, following Foucault, calls the product of these scientific procedures a “docile object”:
It is an object that “behaves” in accordance with a programme of normalization. This does not mean that it fails to resist, or that its recalcitrance does not serve to adumbrate its objective news for science. It is to say that, when an object becomes observable, measurable and quantifiable, it has already become
civilized; the disciplinary organization of civilization extends its subjection to the object in the very way it makes it knowable. The docile object provides the material template that variously supports or frustrates the operations performed on it. Its properties become observable-reportable in reference to the practices for revealing them. If the object was not compliant to such a programme, its attributed properties would be incompletely or “unscientifically” observable.
69
This same civilizing process applies equally to the human body in motion studies, the paramecium under the biologist’s microscope, or the astronomer’s optical pulsars.
70 I would argue that it also applies to the apparatus created or transformed to view or inscribe these phenomena. Motion picture technology also underwent a certain domestication as its image was transformed into acceptable scientific evidence. For the cinematic image to function legitimately as scientific evidence, it must undergo a transformation, a
rendering that is common to all scientific practice and “docile objects.” The army’s flip books, for example, were used to train the soldier’s body, to corral its forces into a productive and useful activity. The books were part of the system of discipline. Yet the very creation of the flip books was also one way of making the cinematic image analyzable by controlling its size, speed, and impact. In the same way, Braune and Fischer took specific, formal steps to domesticate both the human body and their chronophotographic apparatus. The next section will describe these steps in some detail and discuss how they fit into the broader process of representing natural phenomena in science.
BRAUNE AND FISCHER’S THE HUMAN GAIT
The Human Gait appeared as a series of papers published between 1895 and 1904 in the
Proceedings of the Royal Saxon Society for Sciences (Abhandlungen der königlich sächsischen Gesellschaft der Wissenschaften). Although Braune died immediately after the experiments described in their first chapter (perhaps testifying to the exhausting nature of the work), Fischer honored his teacher by making him the first author, and both names have been associated with the studies ever since. Chapter 1 appeared in 1895 and chapters 2 through 6 appeared in 1899, 1900, 1901, 1903, and 1904.
71 Sponsored by the German High Ministry of War, the essays were the first quantitative analysis of human locomotion, and their precision set a standard that is still frequently cited today. That an English translation appeared in 1987—and is still considered an important text in the modern field of human motion studies—testifies to their continued relevance in the science of human movement. In addition to formulating important axioms for this field, Braune and Fischer developed new techniques and instruments for analyzing images. In fact, they are recognized as the originators of analytic, close-range photogrammetry, the science of measurement from photographs.
72
Previous investigators of human motion, such as the Weber brothers, Marey, Muybridge, Carlet, and Hermann Vierordt, were primarily concerned with qualitative, two-dimensional motion studies. That is, their largely descriptive studies focused on motion through only two axes—the horizontal and vertical lift of the human leg, for example. Braune and Fischer, on the other hand, realized that animal locomotion takes place along a
z-axis as well, that is,
sideways. They strove to create, through experimentation and exact measurement, an ideal, three-dimensional model of human movement, something that had not been done up to that point. Several researchers had tried to represent the intricacies of human movement graphically, but their methods lacked the scientific rigor to which Braune and Fischer aspired. Braune and Fischer noted, for example, that the achievements of Muybridge, Anschütz, and Londe
73 “are very important for artists, particularly those who depict people and animals in motion,” but “the use of photography as a scientific research tool and the improvement of cameras to this end are due, above all, to Marey.”
74
The primary difference between studies “important for artists” and those that could be counted as “scientific” was measurement. Muybridge and Londe had in common the instinct for “automatic writing,” that is, some method of creating automatic, mechanically inscribed signs of movement. But such a sign alone would be useless if it could not be held up to ever stricter scientific protocols. Marey’s single-camera setup represented considerable progress over Muybridge’s series of cameras, simply because, for Muybridge’s series method to be successful, “the distances between axes of the cameras, standing side by side, had to correspond to the phases of movement and the different cameras had to be optically similar” (6). This distance depended upon the velocity of the moving body, which could not be known beforehand and is different for each body and each type of movement among the various body parts. Therefore, precise comparison between the pictures was nearly impossible. Yet Marey’s single-camera system had problems as well. “If all the points of a human or animal body moved in one plane during walking or running, these series of pictures would represent not only a one-sided projection but also a true picture of the whole process of movement. However, movement in space has to be taken into account, whereby the centers of all joints describe double curves. Thus, the projection achieved by Marey’s method is insufficient to describe completely the movement in space” (8). In other words, while Marey’s pictures provided information about the horizontal and vertical motions of the entire body during walking, they provided no insight into its sideways oscillations. Braune and Fischer’s contribution, then, was a four-camera system that produced “two-sided” chronophotography, because “two simultaneous photographic exposures of the same movement are sufficient to determine the movement in any direction” (10).
Two-sided chronophotography had its own peculiar difficulties, however. It “requires the cameras to be opened and shut at short intervals at precisely the same time. This requirement can only be achieved using a highly complicated mechanism. Therefore, to interrupt the exposure, we relied not on shutting the camera but on altering the photographic object itself, so that it was possible to dispense with a particular mechanism for shutting the camera” (10, emphasis added). From the beginning, then, Braune and Fischer confronted the dilemma of simultaneously regulating subject and apparatus. This was not an either/or situation; the subject was not completely subdued in favor of an implacable apparatus and method. Rather, both were altered until their properties merged in the representation. That is, features of the body that could be graphically enhanced (such as a limb’s straight line) were coordinated with characteristics of the camera (such as the two-dimensional film plane) to create a usable image. This stage of their experiment, then, was crucial for adapting the body to the chronophotographic image and vice versa.
Braune and Fischer’s alterations included, first of all, highlighting the recruit’s body with a series of strategically placed Geissler tubes—long, thin, straight tubes filled with rarefied nitrogen that, when exposed to an electric current, become incandescent. The recruit wore a black jersey, similar to the one used by Marey, which “provided a dark background for the tubes and permitted better attachment of the tubes to the body” (12) (
fig. 1.1). When fired by a regulated electrical circuit, the flashes of light provided an ideally manageable strobe effect. The black jersey offered not only a dark background for the tubes, it effectively erased all extraneous details—meaning most of the body itself—from the picture and presented only the most graphic qualities of the process under examination. Significantly, Braune and Fischer also took into account the points that were not illuminated (
fig. 1.2).

In some places the Geissler tubes were surrounded by narrow rings of black Japan varnish; these places thereby appeared as short interruptions in the line of light in the pictures. They were located near the ends of the tubes and at the same level as the center of the joint. They therefore marked the corresponding positions of the joints as isolated points of light in the photographs. Similar black rings were also located at the places corresponding to the center of gravity of each segment of the body; the different centers of gravity appeared, then, as black dots on the white lines in the photograph (14).
Generally speaking, Braune and Fischer were “marking” the object in preparation for its representation, a process that is common in scientific rendering practices. In recounting how a “natural” space is rendered into a geometricized workplace, Lynch identified a number of themes or processes. Exploring how representations—graphs, tables, diagrams, and photographs—come to embody the “natural object,” he asks “how science initially determines what is natural on the basis of what its graphic qualities disclose.”
75 “Marking” is a first step toward identifying and cultivating those graphic qualities. Lynch finds that marking occurs in two phases: “labeling” and “upgrading visibility.” In the first phase, the visibility of the object is initially consolidated by first-order techniques, for example, dyeing a cell so that its constituent parts show up under microscopic study. In the second, scientists mark instances that stand out as clear examples, which is a process of selective perception while the project is underway. Braune and Fischer’s Geissler tubes served this dual purpose, then, by both enabling the visibility of the object and making visible only what already had been identified as significant.
The second step common to this rendering process, so closely related to the first as to be only theoretically distinct, is “the constitution of graphic space.” Here a “‘mathematical’ space comes to dwell within the ‘natural’ terrain.”
76 Scientists mark the space of the experiment in such a way that it can be measured or formally structured. In the happy coincidence of anatomy and classification, or the way a specimen is appropriated so that its visible properties are brought together with the graphic qualities of the representational medium, natural objects are prepared for “mathematicization.” In other words, the surfaces of the natural object are brought in line with ideal, geometric properties that can be mathematically useful. The placement of the Geissler tubes illustrates nicely how the protomathematical properties of the human body merge with the graphic qualities of the chronophotographic image to become an intelligible, analyzable, measurable scientific representation.
Braune and Fischer also constituted the graphic space by careful camera placement and superimposition of a coordinate grid. They placed two cameras across from each other and perpendicular to the
x-axis (the recruit’s path) and two other cameras at 30-degree angles to the
x-axis (
fig. 1.3). The two simultaneous exposures (of each side of the body) could therefore determine the position of any given point in three-dimensional space. To ensure that the points on the different exposures were registered exactly in relation to one another, Braune and Fischer created a grid that could be superimposed upon the image, so that the points of light could be matched to an established set of coordinates (see
fig. 1.2).

To enable us to draw the trajectories in a system of tridimensional co-ordinates, after photographing the phases of movement we photographed on the same plate a network of 1-cm squares printed on a glass plate covered with Japan varnish. In order to improve accuracy we built a large wooden frame on which a 1-m square table of co-ordinates could rotate about a vertical axis. This frame rested on four small screws for which four metal recesses were prepared in the floor. The frame and the table of co-ordinates could thus be brought into exactly the same position at any time. (16)
So the wooden frame held a one-meter-square glass grid that could rotate parallel to the plane of each of the four cameras. After the initial exposures of the recruit were finished, Braune and Fischer would cover the cameras, screw the frame into its predetermined spot on the x-axis, and superimpose the grid onto each of the four photographic plates, thereby creating a “graphic space.”
It was also necessary that the different phases of movement be regulated temporally. To coordinate the firing of the strobe effect, Braune and Fischer connected the primary circuit of the induction coil to a large tuning fork (15). They determined that the fork vibrated at a frequency of twenty-six vibrations per second, which meant that between any two phases of movement approximately 0.04 seconds elapsed (16). The frequency of the fork did not matter so much as its regularity.
Connecting the subject to an electrical source also regulated him in a more indirect way. Braune and Fischer sewed long strips of gutta-percha (a substance resembling rubber but containing more resin) into the jersey where the tubes were to be placed to protect the recruit from electric shock. “Perhaps we were overconcerned with regard to the insulation and could have saved ourselves a great deal of work since it usually took us between 6 and 8 hours to dress the experimental subject. However, we thought that the subject would walk naturally if he knew that the electric current, of which so many people are afraid, would not come into contact with his body” (14). Indeed, it would be surprising if the recruit actually did walk naturally. The eleven Geissler tubes were connected in series and powered by a large induction coil in the laboratory. Wires from the coil hung from the ceiling, were draped over a light wooden rod fixed across the shoulders of the recruit (see
fig. 1.1), and connected to the circuit on the body. The recruit could therefore walk freely for about ten meters, “the length of the room necessary for the experiment” (14). Camera placement played a crucial role in the success of the experiment, so it was essential that the subject not waver from a specified path, which the constraints of the wires and the threat of electrical shock certainly ensured.
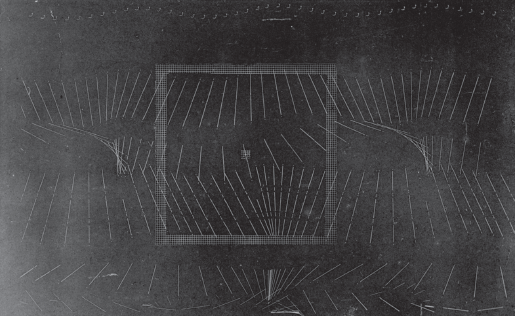
Clearly, then, by the time the exposures were made, the phenomenon of human movement had already been transformed from an unreadable, “natural” object into a regulated, mathematicized process. The photographic image’s unruly detail had also been restrained in a number of ways: the strobe effect reduced the image to only its most graphic, mathematical components; the camera placement and the coordinate grid regulated the space of the experiment; the tuning fork and Geissler tubes marked the temporality of the images. The resulting chronophotographic image, however, was far from self-explanatory (
fig. 1.4). No image is meaningful without another set of interpretive routines.
If they were to create a three-dimensional model from these images, Braune and Fischer needed to measure the distances between the points of light and darkness. Figure 1.5 can only hint at some of the spectacular trigonometric operations involved in determining three-dimensional coordinates from two photographs, operations that I will not gloss in any detail. Generally, however, they used triangulation, the basic principle of photogrammetry, to find the position of any point in space from the bearings of two fixed points a known distance apart. This required, of course, that they take measurements directly from the photographic plates. To accomplish this task, they created an instrument designed especially for this purpose (
fig. 1.6).
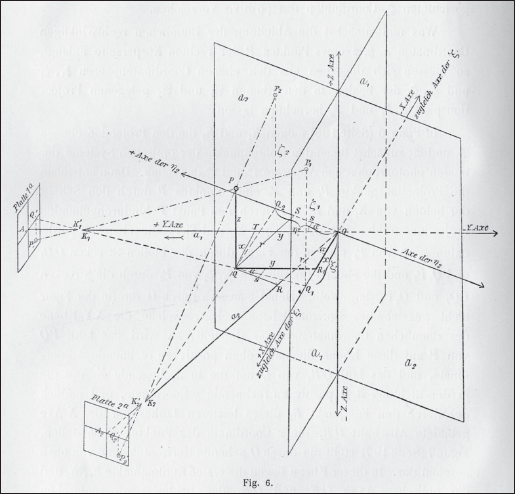
The photographic plate was fixed upon a mobile ring and viewed through a microscope, which could slide along a track and bring any point on the plate into view. The microscope gave a view of both the image on the photographic plate and a ruler placed alongside the plate, thereby allowing easy measurement of the points on the plate (
fig. 1.7).




These measurements for all four views were then collected and, “to avoid an accumulation of data” (!) (43), tabulated for only nine points on the human body: the shoulder, elbow, wrist, hip, knee, ankle, center of gravity of foot, tip of foot, and the point on the head (
fig. 1.8). This reduction of the recorded points to a workable number was the first step in transforming the wealth of data into an ideal, that is, a theoretical model. It should also be noted that the gaps in the tables represent coordinates that for some reason could not be determined (a forearm blocking a point on the hip, for example); likewise, question marks indicate indeterminate data. These statistics, although varying by individual experiments, were consistent over time. “Thus, the results reported in the tables of co-ordinates are valid not only for an individual. They also represent general laws of the movements of the limbs in human gait” (80). At once more than and less than a human body, the numbers provided the principal tool by which Braune and Fischer reconstructed human movement.
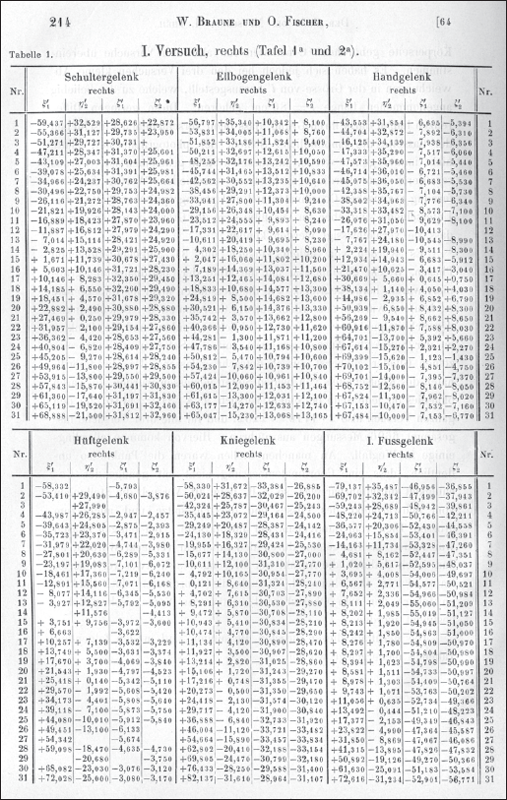
The second step in “polishing” the rough data involved plotting the coordinate numbers onto a graph, essentially recreating the recruit’s movement on another grid (
fig. 1.9). Idiosyncrasies were reduced by the use of straight lines and points. Comparing one of the photographs (see
fig. 1.2) with the graph, we can see clearly how theoretical presuppositions informed the creation of the image. Yet Braune and Fischer maintained, “It must be stressed that the lines drawn in the diagram do not represent the Geissler tubes used for the experiment but the long axes situated inside the limbs” (81). Their coordinates also provided for a series of horizontal, or overhead, views of human movement. Figure 1.10 provides successive overhead views of the movements of the nine sections of the body. The views were separated, of course, to make them easier to read.

Edmund Husserl, writing on “The Origin of Geometry,” outlined how material objects in the real world are identified with idealized geometric forms:
First to be singled out from the thing-shapes are surfaces—more or less “smooth,” more or less perfect surfaces; edges, more or less rough or fairly “even”; in other words, more or less pure lines, angles, more or less perfect points; then, again, among the lines, for example, straight lines are especially preferred, and among the surfaces the even surfaces; for example, for practical purposes boards limited by even surfaces, straight lines, and points are preferred, whereas totally or partially curved surfaces are undesirable for many kinds of practical interests. Thus the production of even surfaces and their perfection (polishing) always plays its role in praxis.
77
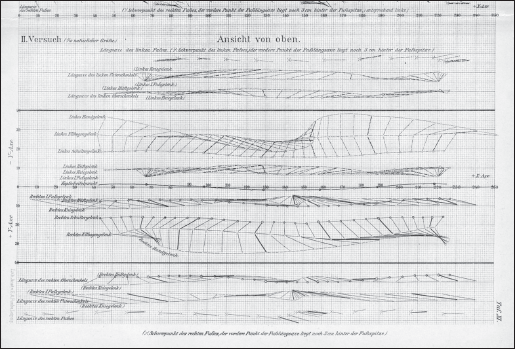
Lynch suggests that we read Husserl’s account “as a description, not of a once-and-for-all historical movement from proto-science to science, but as an account of what scientists do every time they prepare a specimen for analysis in actual laboratory work.”
78 We can see this mathematization, this “polishing,” take place as Braune and Fischer smoothed out the rough edges of their data from table to graph. The body/image became progressively less recalcitrant as the process continued. Like a student who erases the rough pencil sketches and calculations only after finishing the final graph in pen, so Braune and Fischer’s polishing erased the contingent, individual body for a generalized, ideal one. It became an
eidetic image.
Eidos is the Greek word for “idea” or “species,” but Bergson elaborated on this definition:
We might, and perhaps we ought to, translate
eidos by “view” or rather by “moment.” For
eidos is the stable view taken of the instability of things: the
quality, which is a moment of becoming; the
form, which is a moment of evolution; the
essence, which is the mean form above and below which the other forms are arranged as alterations of the mean; finally, the intention or
mental design which presides over the action being accomplished, and which is nothing else, we said, than the
material design, traced out and contemplated beforehand, of the action accomplished. To reduce things to Ideas is therefore to resolve becoming into its principle moments, each of these being, moreover, by the hypothesis, screened from the laws of time and, as it were, plucked out of eternity. That is to say that we end in the philosophy of Ideas when we apply the cinematographical mechanism of the intellect to the analysis of the real.
79
The culmination of Braune and Fischer’s experiment, the final eidetic image, the reduction of real movement to an Idea—arrived at through an application of the cinematographical mechanism—was their three-dimensional model of human movement (
fig. 1.11). With this model, they attempted to reconstruct movement by means of a series of discontinuous images. Their final operation, then, was essentially cinematic.
In this case, science’s successful appropriation of cinema or chronophotography seems to have depended upon the ability to analyze the image and derive translatable information from it. The indeterminate detail of the image needed to be regulated, interpreted, and translated into data that could be mathematicized, tabulated, graphed, and modeled. Quantitative frame analysis, then, has been the traditional means of extracting this information or of disciplining the image. Anthony Michaelis, in his 1955 survey of the history of the scientific applications of motion pictures, declared that “only the quantitative use of cinematography, combined with frame analysis, has produced the maximum amount of research data of which the motion picture film is inherently capable.”
80 In other words, if the image were to be a fully productive member of the scientific community, it needed to be, like the worker, broken down and reconstituted.
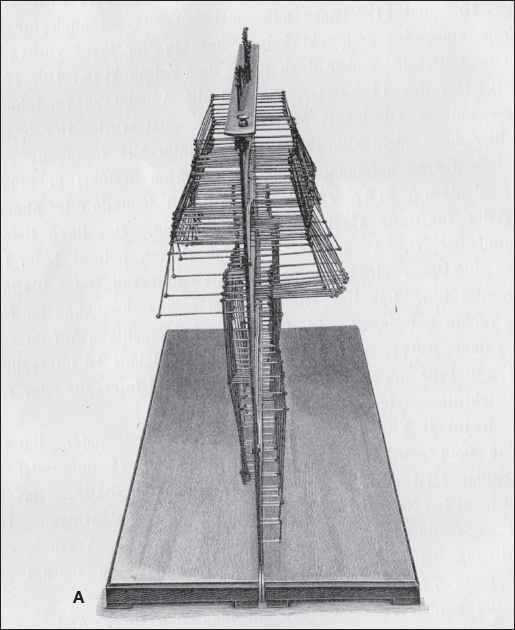

Braune and Fischer’s efforts confirm Bergson’s idea of the cinematic nature of science. In their attempts to chart the movement of the human body over time, Braune and Fischer were not concerned with duration so much as with isolation of particular moments. (They were also very interested in training the expert eye; their three-dimensional model in this respect functions as a guide to what to look for when studying human movement.) Film’s success as a scientific instrument depended above all on its malleability and on the ability of scientists to manage the excessive detail within the image by reconfiguring the apparatus and image through various methods of frame analysis. Braune and Fischer’s example is instructive because it provides a relatively clear view of the work involved in making a malleable and legitimate image. They also provide a good example because their simultaneous work on the image, the apparatus, and the human body replayed certain themes significant to cinema’s relation to science and especially the science of work: the domestication of the object and apparatus, the isolation of physiological functions, and the disassembly and theoretical re-formation of movement and process. Braune and Fischer’s example suggests that chronophotography’s legitimacy as a scientific instrument rested not so much on its ability to record movement through time as its ability to stop that movement—that is, not so much its continuity as its
discontinuity. Above all, the ability to divide processes into ever smaller units appealed to the late nineteenth-century researcher.
Braune and Fischer’s method depended upon finding the geometry inherent in their subject—in this case, the human body—and translating it into mathematical terms. Chronophotography was essential to this operation, because it could isolate the “determinate moments” (in Bergson’s words) of the flux of movement so that the inherently geometric aspects of the body could be highlighted and studied. As Bergson argues,
There is an order approximately mathematical immanent in matter, an objective order, which our science approaches in proportion to its progress. For if matter is a relaxation of the inextensive into the extensive and, thereby, of liberty into necessity, it does not indeed wholly coincide with pure homogenous space, yet is constituted by the movement which leads to space, and is therefore on the way to geometry. It is true that laws of mathematical form will never apply to it completely. For that, it would have to be pure space and step out of duration.
81
Here Bergson is building on his argument that matter, or more precisely form, is a “snapshot view” of reality in transition. Yes, everything is in continual flux, but this constant movement (hence contingency, which, for Bergson, implies liberty) sometimes, to our perception, “relaxes” into a more or less stable shape. So from the perspective of this moment—the “moment” during which the chair I am sitting on appears to be and acts solid and stable, which is a longer or shorter moment, depending on one’s viewpoint—we can map and measure this form and make use of this momentary “interruption” in duration. We can therefore make use of the geometry inherent in form, or what Bergson calls the “mathematical immanent in matter.”
Both René Descartes and Isaac Newton recognized this “mathematical immanent in matter.” Descartes worked toward a mathematical physics when he argued that certain qualities (such as extension, shape, and motion) were more knowable, objective, or certain than other qualities (such as color, sound, heat, and cold).
82 He posited that we could know these “primary” qualities through measurement and ground our knowledge in the more certain world of mathematics. He thereby mapped geometry onto the physical world more thoroughly than his predecessors and opened the way for Newton’s mechanics as mathematical formulations of motion. Classical physics was therefore founded upon matching the material world to mathematics. But, as Bergson noted, the pure form and space of geometry never completely match the matter of the object under study, simply because the object exists in time; in math, the elements of the equation do not age and change. Braune and Fischer’s method indicates the lengths to which scientists must go to reconcile the
duration of the object (however momentary) with the
space of geometry and thereby harness the “mathematical immanent in matter.” Hence Bergson’s conclusion that “there is something artificial in the mathematical form of a physical law” (238).
Yet science succeeds. Bergson offered this explanation: “One hypothesis only, therefore, remains plausible, namely, that the mathematical order is nothing positive, that it is the form toward which a certain
interruption tends of itself, and that materiality consists precisely in an interruption of this kind.”
83 In other words, Bergson recognized a homology between science’s method of arresting time and the momentary interruption of duration that is materiality. Or, to put it another way, science’s tendency to
spatialize time is analogous to the “relaxation” of duration into form and matter. In any case, according to Bergson, science’s success in finding mathematical correspondences depends on a temporal interruption.
Max Seddig’s attempt to confirm Einstein’s theory of Brownian motion is an interesting case study, because his cinematic and chronophotographic method corresponded so closely to important features of the theory.
84 Einstein’s theory, as I will explain later, emphasized a gap or interruption: an elision of the path of particles in favor of two independent but related points along that path. In other words, Einstein stressed the
displacement of particles rather than their actual path. Likewise, Seddig’s experimental method focused on the interval
between filmic exposures, so that two separate exposures represented two independent but related points. Seddig’s results captured more fully than most the specifics of Einstein’s theory precisely because his method was able to accommodate this gap or displacement that previous researchers missed or ignored. Seddig harnessed the inherent discontinuity of the chronophotographic/cinematographic method to create an experimental system that corresponded to Einstein’s important displacement equation. He experimentally created a “temporal interruption” akin to what Einstein had created theoretically, thereby instantiating the spatialization of time and form that Bergson found to be central to science’s success. More than opening up new realms of the visible or even confirming important theories, this use of motion pictures in physics also illuminates certain features and limits of the scientific method as it had been traditionally understood. Indeed, examining Seddig’s experiments alongside Bergson’s critique allows us to see the deep compatibility between this application of motion pictures and the state of the physical sciences at the turn of the century. This section will proceed in the following manner: after a general discussion of the stakes involved in the discussion of atomistic physics, I will explicate Einstein’s theory of Brownian motion as a watershed moment that collected and focused scientific observation. A survey of the experimental attempts to capture or confirm this phenomenon will lead us to Seddig’s case and a close analysis of the connection between his experimental system and Einstein’s theory. Finally, we will return to Bergson in order to ruminate on the homology between film form and the physics of Einstein and Seddig.
Einstein’s theorization of the phenomenon of Brownian motion, for which he assumed the atomic–kinetic theory of matter, is generally considered to be “one of the fundamental pillars (or even the main one) supporting atomism in its victorious struggle against phenomenological physics in the early years of this century.”
85 Throughout the nineteenth century, two approaches to scientific explanation had been in competition in classical physics: the atomic–kinetic theory of heat, on one hand, and on the other, a phenomenological thermodynamics, which assumed that all natural phenomena could be encompassed by the application of Newton’s laws of mechanics, but which did not presume to have access to the ultimate constituents of matter. (This approach to physics is called “phenomenological” because it deals with the description and classification of phenomena while refusing to indulge in any claims about causation.) This approach allowed physicists to describe certain phenomena (such as heat transference) and derive laws (such as the first and second laws of thermodynamics) from observation without explaining
why matter and energy acted in this way. These laws of thermodynamics therefore functioned much like other laws of classical physics, such as Newton’s law of gravity, in that they presumed that phenomena would obey these laws no matter what their size or situation. These laws, in other words, were generally regarded as both scalable and absolute.
The rival approach to explaining thermal phenomena was the atomic–kinetic theory of matter, which started with specific assumptions about the constituents of matter, that is, “that it was discrete, molecular, ultimately atomic, and that heat was a ‘concealed’ form of motion associated with the molecules of a substance.”
86 This theory had the advantage of actually trying to
explain the nature of heat, but it had the disadvantage of being unobservable. While atomic theories of matter date back to the Greeks, many eminent nineteenth-century scientists, such as Ernst Mach and Wilhelm Ostwald, resisted building theories of physics on the supposed behavior of matter consisting of particles so small that they were invisible.
87 If advocates of the kinetic theory were to succeed, they would need to deduce the existence of these particles from their effects. Einstein and others theorized that these effects could indeed be deduced mathematically from the random fluctuations in an observable system, such as a container full of a certain kind of gas. These random fluctuations would not occur, by definition, if the absolute laws of thermodynamics held. In other words, the proof of the real existence of molecules was tied to proving that classical thermodynamics was true only in a statistical—not absolute—sense. And at the turn of the century, the atomic theory of matter and its statistical correlation were far from universally accepted. The theory and confirmation of Brownian motion was a crucial victory in this struggle.
Brownian motion, the irregular motion of microscopic particles suspended in fluid, had been known long before Scottish botanist Robert Brown described it in 1828, when he turned his microscope to cytoplasmic granules extracted from pollen. His achievement was “to show that the motion could not be attributed to any supposed vitality of the particles themselves, since all kinds of inorganic as well as organic substances behaved similarly.”
88 This meant that the cause of the movement had to be external to the particles themselves. There were a variety of attempts to explain this phenomenon, but it was not until the close of the century that French physicist Louis-Georges Gouy suggested that Brownian motion constituted a clear demonstration of the existence of molecules in continuous, albeit random movement.
89 However, Gouy did not work out any mathematical theory that could lead to experimental confirmation. This was Einstein’s contribution in 1905. Not that previous scientists hadn’t tried. But the attempts to clarify the phenomenon experimentally were hindered by the lack of agreement among the observations. Researchers simply could not agree on the principal features of Brownian motion. Not only were the movements too quick and irregular to submit themselves to steady observation, but researchers could not concur on even such basic presumptions as whether the movements were dependent on the temperature of the fluid. This tangle of confusion indicates, to Roberto Maiocchi at least, “how difficult it is to make a meaningful and conclusive scientific ‘observation’ and, as a result, how any inductivist conception, which claims to start from an empirical base in order to then construct theories of some importance, is unsustainable.”
90
Photography and cinematography offered at least the possibility of creating an “objective” record that could be compared with previous and current descriptions of Brownian motion. But again, without sufficient technical means and a theory to guide them, this hope was fool’s gold. Take, for example, the case of Austrian scientist Felix Exner, who attempted “to measure the size of the particles and their speed” via direct photographic exposure.
91 Unfortunately, the photographic plates at the time were not sensitive enough to register the small amount of light coming through the microscope lens. Undaunted, he observed the movements and traced them
manually onto a blackened photographic emulsion, projected these traces onto a screen, measured the distances between the points, and divided by the observation time. “Of course, the values are not very exact,” he admitted.
92 Indeed. Exner was interested in establishing the numerical relationship between the temperature of the fluid and the velocity of the suspended particles. His article was the first attempt to quantify features of Brownian motion that previously had been described only qualitatively. Even though his results were the most accurate studies to date, given his method, it is not entirely surprising that they were not decisive. But he did spotlight the need for systematic observation and measurements and the role photography could play in this process.
But previous observation had very little impact on Einstein’s formulation of his theory. The jumble of conflicting observations was of limited use. Einstein was not interested primarily in generating a theoretical explanation of a puzzling phenomenon. Instead, he saw Brownian motion as an observable system that could conceivably resolve the debate between phenomenological thermodynamics and the atomic–kinetic model. Specifically, he derived equations governing motion based on the assumption of random molecular movement and then argued that their confirmation would demonstrate the existence of molecules and also show that the laws of thermodynamics did not apply absolutely to particles of molecular dimensions. In his 1905 paper on the topic, Einstein indicated both the inadequacy of previous observations and the stakes involved for future observations:
In this paper it will be shown that according to the molecular-kinetic theory of heat, bodies of microscopically visible size suspended in a liquid will perform movements of such magnitude that they can be easily observed in a microscope, on account of the molecular motions of heat. It is possible that the movements to be discussed here are identical with the so-called “Brownian molecular motion”; however, the information available to me regarding the latter is so lacking in precision, that I can form no judgment in the matter.
If the movement discussed here can actually be observed (together with the laws relating to it that one would expect to find), then classical thermodynamics can no longer be looked upon as applicable with precision to bodies even of dimensions distinguishable in a microscope: an exact determination of actual atomic dimensions is then possible. On the other hand, had the prediction of this movement proved to be incorrect, a weighty argument would be provided against the molecular-kinetic conception of heat.
93
Einstein tackled the big problems; his equations would either prove or disprove the atomic theory of matter.
Because he did not invoke previous studies or conduct experiments of his own, Einstein’s vision of Brownian motion was not empirically based; instead, “Brownian motion” was for him a system that he had mathematically pared down, even created, which could then be observed and measured.
94 Einstein did not so much
describe Brownian motion as
manage it mathematically, thereby providing researchers with the theoretical guidance they needed, giving them mathematical pointers so that they would know what to look for. As Maiocchi notes, “Only when Einstein had constructed,
independently of the experimental accounts, a sufficiently articulated theory, did the experimenters know
what had to be observed and only after this theoretical clarification did the observations turn out to be conclusive.”
95 Einstein’s theory, more than any technology, corralled subsequent observations into a stable of usable data.
Of course, before they could be so guided, the researchers first had to
understand Einstein’s work, and this was far from a foregone conclusion. As Mary Jo Nye remarks, Einstein’s 1905 and 1906 papers included mathematical derivations that “were certainly beyond the ken of even the more precocious experimentalist.”
96 The novelty of Einstein’s equations lies in his emphasis on the
displacement of the particles rather than their actual path or velocity. As noted earlier, Einstein was interested in proving the real existence of molecules and also in demonstrating that thermodynamics was true only statistically and not absolutely. These two goals were intimately related, in that any kinetic theory of molecules had to incorporate a thorough understanding of statistical mechanics. The observed properties of a gas, for example, depend on the
average behavior of its molecules. But in any system that is sufficiently random—and the model of gas as a system of molecules assumes this randomness—there will be fluctuations from this average behavior. If such fluctuations were large enough, then they would put into doubt the stability of the measured properties and thereby raise questions about classical thermodynamics. In Brownian motion, Einstein had found a system of observable fluctuations that could prove the existence of molecules.
97
These fluctuations were expressed in his equations as the mean square displacement (λ
x)
2 of a particle in any given direction in any given time interval. Einstein realized that particle velocities were so great that they would never be observed directly and therefore could not be measured accurately. So his solution was to factor the displacement of the particles.
98 Maiocchi explains:
While previously the attempt had always been made to estimate the length of the trajectory actually traversed by a particle, Einstein’s theory deals with the
displacement effected in a given time, i.e., the intervening distance between the points of departure and arrival,
independently of the path followed. This is a change of radical importance because it changes completely
the object of the observation: it is no longer a matter of trying to measure the velocity of the Brownian movements (obtained by dividing the length actually traversed during the observation time by the time itself), but of a different quantity.
99
This solved the problem that had bedeviled researchers in their previous attempts to describe the phenomenon, as Stephen Brush argues:
Einstein showed that there is no possibility of observing this velocity…. Hence any attempt to measure the “instantaneous” velocity of particles in Brownian movement will give erratic and meaningless results. It is for just this reason that all the efforts of the experimentalists … had failed to lead to any definite conclusion about the average speeds of suspended particles. They were simply measuring the wrong thing until Einstein pointed out that only the ratio of mean square displacement to time could be expected to have any theoretical significance.
100
Previous experiments, such as Exner’s in 1900, had focused incorrectly on the
path of the particles. Since the invention of the ultramicroscope in 1903, researchers had turned to Brownian motion with renewed interest, further spurred by Einstein’s paper in 1905.
101 Some followed Exner’s lead in measuring the actual paths, not catching Einstein’s insight. French physicist Victor Henri’s results, for example, published in 1908, are notable in that he too used cinematography to record particle movement and to trace particles’ actual paths.
102 His results did not agree with Einstein’s theories and momentarily cast doubt on Einstein’s equations. But it soon became clear that experimental error negated Henri’s results.
103 The winner in the Brownian motion sweepstakes was Henri’s countryman Jean Perrin, who ran a series of experiments that were also published in 1908. Initially, Perrin paid little attention to Einstein’s papers, but when he examined them more closely after 1908, he realized that his experiments confirmed Einstein’s predictions. Further work by Perrin produced the most persuasive experimental confirmation of Einstein’s theory; after that, virtually no one in the scientific community doubted the existence of atoms, and in 1926 Perrin won the Nobel Prize for his efforts.
104
Which brings us to Seddig. Even though Seddig achieved results comparable with those of Perrin, they were, according to Nye, “neither as accurate nor as convincing.”
105 While Seddig is therefore little more than a footnote in the traditional accounts of this moment in the history of science, he did discover an important homology between an element of his experimental method (cinematography and chronophotography) and an aspect of the new theory of physics (displacement). Like Exner, Seddig was interested in verifying the dependence of particle velocity on the temperature of the fluid. And like Exner, Henri, and others, Seddig first attempted to record the actual paths of the particles via photographic exposure through an ultramicroscope. He hoped that with long exposures of the particles, the traces left on the photographic plates would correspond to the paths:
It seemed obvious to attempt to photograph the moving particles, which show up in the ultramicroscope as luminous points, on a stationary plate for a certain exposure time (around one second). The luminous, moving points should then sketch black lines on the plate, which should correspond to the horizontal path covered during this time. The lengths of the resulting curves obtained at
different temperatures but during
identical intervals should then stand in the reciprocal relation predicted by the theory.
106
But again, as with Exner, this method failed due to the weak light from the ultramicroscope and the relatively insensitive photographic emulsions available at the time.
However, after this failure, Seddig employed a regular microscope, which provided stronger light and therefore better exposures, and an attached cinematograph. Again, like Henri, he tried to record the movements of the particles cinematographically. As he notes in his first presentation of his work, “A cinematic method with a precision camera gave some results that were also sufficiently precise.”
107 While his modified cinematic apparatus seemed to achieve a greater degree of exactitude, he was eventually dissatisfied with the instability of the cinematic image in his efforts to trace the paths. So his final results were determined with the use of an ultramicroscope and a series of multiply exposed photographic plates (
fig. 1.12). But while experimenting with cinematography, Seddig came upon the solution to a vexing problem. He had noticed that the strong light required to illuminate the particles also heated the suspending fluid, generating a temperature increase that could not be predicted and that therefore jeopardized his data. He minimized this problem by substituting an intermittent light source for a continuous one.
108 The lamp fired two successive flashes one-tenth of a second apart, while the camera was rigged with a system to measure exactly the time interval between the flashes (
fig. 1.13). The light source moreover was projected through a series of cooling and polarizing filters and reflected off a mirror into the microscope, which was attached to the camera. The camera was attached to an electrical circuit so that with each exposure the circuit would open and close, creating an electrical spark that was graphically recorded on a rotating drum covered with blackened paper. The graphic record served as a control for the frame rate so that the interval between exposures could be measured precisely.
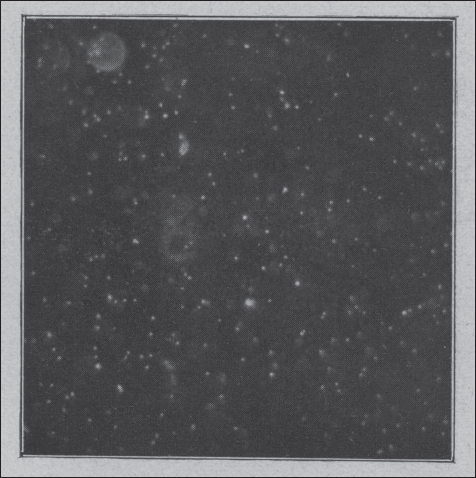
With this setup, Seddig could record the movement of any given particle, but not completely: the intermittent flash recorded only two points along that highly irregular path (for an example, see
fig. 1.14)—say, point
A1 and point
A2. The path of the particle between these two points, which occurred
between the frames, left no trace and was therefore experimentally elided. All that could be measured was the straight line between the two points—
which was exactly the empirical translation of Einstein’
s displacement equation. In other words, Seddig had fashioned an experimental method that corresponded to (and therefore partially confirmed) Einstein’s theory. Einstein praised Seddig’s efforts, even if he was a bit befuddled by Seddig’s method: “I have read Seddig’s paper. He has done it very well. I cannot quite make head or tail of his descriptions of the results.”
109
Why was “displacement” so crucial to Einstein’s theory? Because it worked around one of the major objections to the kinetic theory: the disparity between theoretical velocity and observational velocity at the molecular level. As Polish physicist Maryan Smoluchowski pointed out, experimental observation of particle velocity would never correspond to the theory, because theoretical velocity was simply not measurable by observational procedures: “What we see is only the mean position of the particle, driven 10–20 times a second, each time in a different direction, by that velocity. Its center will describe an unpredictable zig-zag path made up of straight lines
much shorter in length than the size of the particle. Its displacement becomes visible only when the geometric sum of these lines is raised to an appreciable value.”
110 Jean Perrin put it in another way:
The apparent mean speed of a grain during a given time varies
in the wildest way in magnitude and direction, and does not tend to a limit as the time taken for an observation decreases, as may easily be shown by noting, in the camera lucida, the positions occupied by a grain from minute to minute, and then every five seconds, or, better still, by photographing them every twentieth of a second, as has been done by Victor Henri, Comandon, and de Broglie when kinematographing the movement.
111
That is, the particle, buffeted randomly by molecules, constantly moves ever so slightly in a variety of directions—these zigzags, smaller than the particle itself, cannot be observed and therefore measured or empirically confirmed. With the reduction of the equation to a question of displacement without direction, velocity (speed + direction) was no longer an issue.
Thus the gap. Einstein’s theory elided the actual path of the particles over time, erasing it from the equation, thereby creating a theoretical interruption (à la Bergson) that was matched by Seddig’s cinematic interruption. By focusing on the dark space between the frames—or, chronophotographically, between exposures—Seddig’s method mimicked Einstein’s theoretical attempt to erase the space between two points along a particle’s unpredictable path. The gap between exposures was long enough to allow Seddig to measure the displacement of the particle from point A1 to point A2. If it had been too small a gap, Seddig would have traced (erroneously) the path of the particle, as Henri did. Seddig therefore found in his apparatus a specific use that serendipitously suited his needs.
Seddig’s application of photography, chronophotography, and motion pictures to the problem of Brownian motion represents a particularly resourceful partnership between film and physics. We could leave it at that and still note with satisfaction the especially neat fit of technology and theory that this application evinces. Seddig’s techniques matched certain elements of Einstein’s equations and while lacking the precision and expertise of Perrin’s experiments, still counted as partial confirmation. But this correspondence of technology and theory also testifies to Bergson’s insight into the deeper connection between motion pictures and science. If science’s success in finding mathematical correspondences takes advantage of what might be considered a “temporal interruption” in the constant flux of becoming—a “temporal interruption” we call “form”—by finding the mathematical, atemporal line of geometry in that form, then the scientific use of motion picture technology often matched this process by creating its own temporal interruption—the space between frames, for example—and finding mathematical correspondences to the resulting form. The temporal character of motion was therefore reduced to a series of static, discrete, two-dimensional images; time and motion were then “understood” by the measurement of differences between them. Motion pictures, in this application, reinforced the tendency to mistake the category of space for the category of time.
112
Furthermore, the spatialization of time allows us to conceive of time as reversible. Our felt experience of time is that it is irreversible, but our experience of space is that we can pursue it in any direction. So when we represent the flow of time as displacement along a homogenous axis, “Nothing prevents us in this abstract representation,” as French physicist Louis de Broglie remarked, “from supposing that we may reverse the course of time, contrary to the most certain property of real duration.”
113 Likewise, a mathematical equation also implies this equivalence and reversibility. As Suzanne Guerlac explains, “In geometrical terms, if we depict a movement from left to right, we can reformulate it as passing from right to left. In algebraic terms, equations are commutable.”
114 But when we conceive physical processes as reversible, we might fail to consider certain essential properties of real time, namely its directionality. Bergson, of course, argued for a dynamic ontology of irreversible time so that important properties of duration do not escape our understanding.
115
By erasing the direction of the particle—whether the movement is from point
A1 to point
A2 or from point
A2 to point
A1 is irrelevant for the purposes of the theory—Einstein’s equations implied a temporal reversibility that was also mimicked by Seddig’s use of photographic and motion picture technology. In more than one way, the scientific use of film (especially in the physical sciences) was mathematical; in Seddig’s hands, film functioned mathematically. How was this accomplished? Three aspects of this kind of application—the use of a flash, the use of the frame, and the form of the celluloid strip—combined to create a formal, almost geometric support for the mathematical rendering of the phenomenon. Seddig used his flash as an intermittent light source that would not raise fluid temperature as much as constant illumination. But the flash also immobilized the particle by reducing exposure time to an instant and the spatial fullness of the phenomenon to a two-dimensional position. The flash focused both time and space to the sharpness of a point.
116 Furthermore, the regularity of the flash created a series of equivalent points—
A1,
A2,
A3 …—each of which corresponded to a single frame. The frame, in other words, determined the boundaries of the event, both spatially, in that the event took place within the field of the frame, and temporally, in that the frame represented a unit of time. So the flash and the frame reduced, limited, and focused the fullness of the event to a series of separable, measurable points—which is to say that the technology accommodated a mathematical understanding of the phenomenon.
While the photographic series is not strictly commutable—one cannot rearrange the order of the units and still obtain the same result—it is reversible, in that [
A1,
A2,
A3 …] is mathematically the same as [ …
A3,
A2,
A1]. In this respect, the celluloid strip itself functioned as a line, as a trajectory of completed motion. Bergson distrusted the representation of movement as a line, because such a rendering confuses movement as we experience it—that is, as something indivisible—with our imaginative recreation of that movement as a series of stages or points. More precisely, it confuses movement with the line traversed; it conflates what is happening right now with what just happened. The path is past, while movement exists in the moment. We may divide the path into points, but these points have no reality outside of the line drawn. Bergson argued that the difference is simple: “At a stage we
halt, whereas at these points the moving body
passes.”
117 We therefore mistake immobility for mobility, discontinuity for continuity, and past for present. Representing motion as a line, especially one that predicts future motion, is an illusion, which rises from the conception that “motion,
once completed, has deposited along its course an immobile trajectory on which one may count as many immobilities as one wishes. From this, one concludes that motion deposits at each instant a position with which it coincides.”
118 In truth, however, no such deposit occurs, because movement itself does not halt; otherwise it would not be movement. But motion pictures reinforce this illusion, not so much through the projected image, which, as Gilles Deleuze pointed out,
119 is movement, but through the celluloid strip of individual frames. What is an exposed film frame except a “deposit” of a position coinciding with movement? And just like a line, the series of immobile instants on the celluloid strip is completely reversible. Bergson might therefore have argued that cinematic exposure itself is a category mistake.
Toward the end of his dissertation, Seddig remarked on the close similarity of Brownian motion and cell movement, calling for further investigation into the relation between temperature fluctuation and “biological motion” that might lead to an understanding of the deeper “causes of movement itself.”
120 Even if this particular research program never really caught on, in a way it points to the interesting history of cooperation between biologists and physicists during the early days of microcinematography. The precise date of the first moving pictures taken through a microscope has not been recorded, but Marey devotes a chapter to the technique in his 1894 book,
Le mouvement.
121 Marey’s experiments were continued by various disciples, most of them working at the Institut Marey in France. Lucien Bull, for example, is known best for his high-speed cinematography of fast-moving objects, which was an important technique in physics for research on surface tension phenomena. In 1903 he helped biology Professor Antoine Pizon microcinematically record the multiplication of a colony of
Botryllus, or sea squirts.
122 Another disciple of Marey and a pioneer in the field, Charles François-Franck, adapted Marey’s apparatus and made chronophotographic plates of a variety of biological phenomena from 1902 to 1908.
123 Lucienne Chevroton, who worked at the Collège de France alongside François-Franck, similarly added a motion picture camera to a microscope apparatus in 1909 to record microcinematographic investigations of a sea urchin egg.
124 François-Franck and Chevroton offered their apparatus to their colleague Victor Henri, a physicist at the Collège de France, who used it to record Brownian motion, as we saw in the previous section.
125 He, in turn, may have introduced it to Jean Comandon, who during the early years became the most successful microcinematographer of cell movement.
126 Lucien Bull also helped outfit Swiss biologist Julius Ries with a Lumière microscope–cinematograph combination for his research on the fertilization of sea urchin eggs.
127 As early as 1900, with the help of Charles Gaumont, French physicist Henri Bénard used cinematography to study dynamic, convective systems—specifically, the spontaneous formation of inorganic matter into hexagonal shapes in a heated liquid—and went so far to comment on the analogy between this type of motion and biological phenomena.
128 In Germany, physicist Henry Siedentopf, who ran Carl Zeiss’s optical laboratory in Jena and collaborated with Richard Zsigmondy on the invention of the ultramicroscope in 1903, actively used motion picture techniques to investigate crystallography.
129 Siedentopf also helped Heidelberg biologist Hermann Braus with his cinematic investigations of tissue culture, thereby continuing this trend of cinematographic collaboration between biology and physics.
This cooperation between physicists and biologists interested in adapting motion picture technology is not surprising, given that investigators in both fields had set out to study motion as a way of answering difficult questions about causality. Brownian motion, as we have seen, functioned as an observable system that could provide clues to the constituents of matter itself. Biologists similarly searched for observable, temporally continuous systems in which the analysis of motion could resolve previously intractable disputes about the processes governing the organism. Braus, a morphologist and experimenter, used motion pictures for precisely this purpose.
130 His case is interesting because it illustrates the close formal relation between cinema and biology at the turn of the century; motion picture technology not only helped solve evidentiary problems in biology, but the introduction of cinematic means into experimental technique matched broader changes in the discipline itself. Furthermore, Braus’s use of motion pictures demonstrates especially dramatically cinema’s
rhetorical power in science; Braus altered his viewpoint in a dispute because of what he witnessed in a filmic record. This section will place Braus’s cinematic contribution in the context of certain broader debates and experimental trends in the discipline of biology.
131
The dispute in question—between Braus (
fig. 1.15) and American experimenter Ross Granville Harrison (
fig. 1.16)—concerned the development of nerve fibers. Braus and Harrison were not the only figures in the debate, which had been brewing since the mid-nineteenth century. The problem was this: while it was clear that nerve fibers grew, it was not clear
how they grew. Did a fiber grow from one cell or from a chain of cells? How was the connection between fibers and tissues established? Did the nerve grow from the center to the periphery, or was the connection already there in the embryo? The disputants offered at least three theories. Many believed that the formation of the nerve required the participation of many cells, not just one. This was known as the “multicellular” theory of nerve development. Others, such as Braus, maintained that nerve fibers had already been present in embryonic form and that as the organism developed and cells grew and multiplied, the nerves stretched across them, making bridges of nerve fibers that differentiated as they became functional. This was the “protoplasmic bridge” theory. Still others, such as Harrison, argued that nerve fibers grew outward from a single nerve cell into the interstices between other cells. This became known as the “outgrowth” theory, and most biologists would come to agree, but not until around 1930, that this theory was the most accurate and persuasive depiction of nerve fiber development (
fig. 1.17).
132
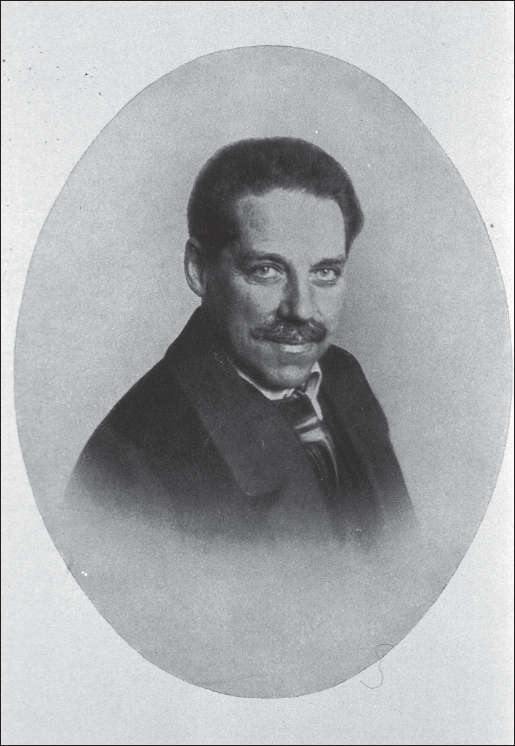
Courtesy of the National Library of Medicine
Courtesy of the National Library of Medicine
From Ross G. Harrison, “The Outgrowth of the Nerve Fiber as a Mode of Protoplasmic Movement,” Journal of Experimental Zoology 9, no. 4 (December 1910): 787–846
There were a number of reasons why investigators in the early twentieth century could not agree on this topic, including prevailing concepts of the structure of the nervous system. But a large part of the problem derived from contemporary methods of observation. As Harrison complained in 1906, “Prior to the year 1904 all attempts to solve these problems were based on observations made upon successive stages of normal embryos. When one compares the careful analyses of their observations, as given by various authors, one cannot but be convinced of the futility of trying by this method to satisfy everyone that any particular view is correct.”
133 That is, researchers attempted to observe the growth of nerve fibers by slicing, staining, and mounting successive stages of an embryo, which were then viewed through a microscope. This technique had long been dominant in biology and developmental embryology.
134 Crucial questions about the origin, direction, and character of growth had to be inferred from what was effectively a series of still images.
Because investigators basically had to speculate about any movement taking place between images, disputes over interpretation were common. Among the most famously frustrating was the debate between Santiago Ramón y Cajal, who subscribed to the outgrowth theory, and Hans Held, who held onto the protoplasmic bridge theory. Susan Billings explains, “Cajal claimed that
Plasmodesmen were artifacts of Held’s preparative method, while Held, in turn, thought perhaps pale staining or alcohol fixation prevented Cajal from seeing them. The impression one might get from reading their papers is that Cajal and Held were discussing vastly different material.”
135 Yet when Cajal visited Held’s laboratory in Germany, he said that he “had the pleasure of examining Held’s excellent preparations. Just as we expected, they are very successful, and to our great surprise, they show very much the same picture as ours.”
136 As with Brownian motion, researchers were unable to agree on what they saw in what were nearly identical preparations. (Indeed, this is another example of the influence of theory on observation.)
The histological technique of slicing, solidifying, staining according to type, and then mounting the preparation on a microscope slide had been the foundation of cell research up through the nineteenth century. It served biology well; as Hannah Landecker notes, “the technique of staining, by suspending the cell in time, freed the experimenter from the temporal exigencies of a living subject.”
137 Precisely because the subject did not move, the researcher could examine the preparation at his or her leisure. “Cellular movement, or lack thereof,” Landecker continues, “was a matter of inference when using static representations, the hardened moments preserved in histological specimens. It was about inferring what was happening in the spaces between the sequential slices of preserved moments.”
138 Indeed, perhaps because this method was so dominant, movement itself was not important to biology’s research questions—one could even argue that biologists’ questions were often tailored to their technique. However, once questions of movement and growth could not be answered, new techniques were required; alternatively, we could argue that once the new techniques were available, new questions came to the foreground.
139 Physicists had solved this problem of observation and interpretation in the case of Brownian motion by emphasizing the gap or discontinuity, thereby excising from the theory that which was not observable. Biologists could not do this, however, because their ostensible object of study was continuity itself. So they had to develop techniques that would allow them to study cell movement directly.
Harrison believed that “the only hope of settling these problems definitely lies, therefore, in experimentation.”
140 In 1905, Braus approached these questions experimentally by transplanting limbs of very young tadpoles to various areas of other tadpoles’ bodies. He then examined the nerves in the transplanted limbs, finding evidence in favor of the protoplasmic bridge theory.
141 Harrison repeated these experiments and came to completely different conclusions. In fact, Harrison argued, “The same facts [in Braus] may be interpreted quite readily, if not more so, in accordance with outgrowth theory. The experiments do not approach the problem directly enough to determine questions of histogenesis [the origin of the nerve fiber], and there are too many loopholes left to permit a rigid proof.”
142 What was needed, apparently, was a means of isolating the nerve cell itself so that the growth of the nerve fiber could be observed directly. In fact, this is exactly what Harrison did in a now-famous experiment that he first described in a June 1907 announcement:
The immediate object of the following experiments was to obtain a method by which the end of a growing nerve could be brought under direct observation while alive…. The method employed was to isolate pieces of embryonic tissue, known to give rise to nerve fibers…. The pieces were taken from frog embryos about 3 mm. long…. After carefully dissecting it out, the piece of tissue is removed by a fine pipette to a cover slip upon which is a drop of lymph freshly drawn from one of the lymph sacs of an adult frog. The lymph clots very quickly, holding the tissue in a fixed position. The cover slip is then inverted over a hollow slide and the rim sealed with paraffin. When reasonable aseptic precautions are taken, tissues will live under these conditions for a week and in some cases specimens have been kept alive for nearly four weeks. Such specimens may be readily observed from day to day under highly magnifying powers.
143
By successfully maintaining a tissue specimen outside of the body (known as “in vitro,” whereas the state of tissue inside the body is known as “in vivo”),
144 Harrison initiated the technique of “tissue culture,” a biological method whereby fragments of tissue from an organism are transferred to an artificial environment in which they can continue to survive and function in some form. With the cell population thereby isolated, the researcher could better examine and manipulate cell behavior. The histologist could observe the growth of the cell directly under the microscope and even slow down or accelerate that growth by manipulating the environment. Investigators from all quarters immediately took notice. Within the next few years, the literature on tissue culture exploded; its most famous experiments were those conducted by Alexis Carrel and Montrose Burrows at the Rockefeller Institute, where they managed to keep the culture from a chicken embryo alive for decades.
145
This method also presented a major break with traditional methods of visualization in biology.
146 Not only did the in vitro experimental technique offer a different way of isolating the cells, but this form of representation also replaced the discontinuous form of representation then common to biology (for example, sequential slides, illustrations of stages of development, still photographs) with temporal continuity (movement, growth).
147 Tissue culture allowed the researcher to observe movement in time; there still remained the challenge of representing this movement, of course, given the traditional means (slides at conferences or illustrations in texts and journals). Harrison’s experiment also showed fairly conclusively that the nerve fiber grew from a single cell. It demonstrated that the fiber could and did grow without the help of other cells and that nerve growth did not require the presence of preformed protoplasmic bridges. Even so, biologists such as Hans Held challenged Harrison’s results for years afterward, maintaining that the nerve fiber grew on its own in vitro but grew with the aid of bridges in vivo.
148
So in September 1911, Braus’s demonstration before the Society of German Natural Scientists and Physicians spoke to both the interest in the new technique and the enduring controversy about nerve fiber development.
149 His demonstration also addressed new challenges in representation in biology, in that he used motion pictures to depict these two issues. At the start of the talk, Braus discussed the still-new technique of tissue culture as Harrison and Carrel had developed it to that point. He then noted, “I thought that many of you would be interested in seeing the phenomena of growth and movement in these cultures, if not in a live culture, at least via cinematographic images. I have films that were made in order to study the more detailed movements in the cultures of my many preparations, in which the transformations were too fast or too slow to be observed in the objects themselves.”
150 This offering to his colleagues at the conference deserves further examination, because it indicates two ways in which film had a crucial role in the experiment.
First, Braus used film to
communicate the results of his work. In this respect, the medium of motion pictures functioned as one of any number of forms of communication that describe or depict experimental procedures so that the (expert) reader can judge the validity and value of the project. Some have called this expert communication and evaluation “virtual witnessing”; in the modern era, when there is no way to inspect or replicate every experiment personally, the significance of such reports for the dissemination and acceptance of new knowledge cannot be underestimated.
151 In this early example, film promoted “virtual witnessing” in an important new way, not only because film made the cultures present to the expert witnesses in a more than virtual manner but because it was portable and repeatable in a way that live cultures were not.
Second, Braus used film as a substitute for his object of study (the tissue from a frog’s heart). He made the films to study movements that were “too fast or too slow to be observed in the objects themselves”; his films were therefore the object of his analysis. In fact, the capabilities of motion pictures so closely matched the capabilities of tissue culture that they became an experiential substitute for the technique. That is, the projected image moves and can thereby present movement or growth, but film was also, like tissue culture, fragmentary and easily malleable, both temporally and spatially. If the histologist could isolate, dissect, transplant, graft, and retard or accelerate the growth of cells, then the cinematographer could match these actions perfectly by framing, cutting, splicing, editing together, and slowing down or speeding up the film itself. (Of course, motion pictures were quantitatively different than tissue cultures because of the ease with which images could be multiplied or accelerated.) In this respect, Braus’s use of film became what we might call a “virtual experiment”—manipulation of the film could isolate and analyze aspects of the tissue that could also be manipulated, isolated, and analyzed in the technique of tissue culture itself.
Braus then described the star attraction of his films: a beating heart extracted from a frog embryo and maintained in culture for days. That achievement was in itself noteworthy, but Braus was interested in proving something more: “As far as I can tell, observations about the
growth of organs in vitro are not addressed in the literature.” That is, he wanted to demonstrate that specimens actually grew in culture, they did not merely survive. So he used staining techniques and time-lapse cinematography: “To prove that the culture, whose pulsation I’ve shown, is actually growing, I have filmed isolated, stained cells from the culture at greater magnifications. The exposures occurred, on average, every 10 minutes over the course of 10 hours. Here in the projection they have been compressed into just a few minutes, so that we can see the movements of the stained cells unfold rapidly, although in reality they are very slow.”
152 The stains allowed Braus to distinguish the movement of the cells and their changing form as they grew and multiplied. His films thereby functioned not just as a substitute for the culture, but as a new form of evidence.
This is a particularly potent example of the power of cinema to present evidence forcefully, because the cinematic record of his experiments with tissue culture caused Braus to reverse his position on the origin of nerve fibers.
To supplement this, I am showing images of growing nerves, in which—just as with the mesoderm cells—many of these growth phenomena can be observed under the microscope
directly. The nerves are of particular interest because of the
in vitro heart movements. At the time of extraction and the end of the experiment, the heart structure had no ganglia cells…. But from our experiences with nerves grown
in vitro, we can rule out the claim that actual nerves originate in any other way than from the central neuroblasts. I want to discuss here objections that could be raised against this conclusion, reached by Harrison based on his preparations, which prompted me to verify his findings. This investigation has persuaded me that Harrison is basically correct.
153
Having witnessed these processes for himself through his filmic record, he was able to declare that “only the isolation of neuroblasts [the nerve cells] gives total certainty; the nerve fiber grows out of the neuroblast like a mold grows out of an isolated spore.”
154 Braus went on to defend Harrison against the objections of other colleagues in the field, with whom he previously agreed.
So Braus also used film as a
confirmation of Harrison’s theory. Braus’s motion pictures were more than a demonstration; they
enacted the technique and Harrison’s theory of growth. Harrison posited the nerve cell fiber as a plant-like “outgrowth” rather than a differentiated element of a multiplying mass. He argued that nerve fiber growth moved forward incrementally, outwardly, and linearly. Likewise, Braus’s time-lapse technique in this instance—with its statistical sampling of time through regular exposures and its amplification of the forward push of incremental growth—enacted and insisted on an incremental, regular, mathematical, and resolutely
linear theory of growth. In other words, this set of techniques (the frame’s isolation of the tissue, the temporal manipulation of growth, and the magnification and projection of the image) enacted, amplified, and confirmed Harrison’s theory of growth and technique of tissue culture with incomparable rhetorical power. While cell growth, when illustrated by sequential images (such as slides), could have been seen as reversible and thus disputable when direction was at issue, the temporal continuity of the cinematic record
pushed the observer (including Braus) to a particular conclusion—that the growth of the nerve fiber happens in
this way. If other scientific uses of cinema try to stop time, this application stressed duration and the teleology of growth. As a means of visualization and as an experimental tool, this application of motion pictures precisely matched broader changes in experimental technique and representation in biology at the turn of the century.
As we can see, motion picture technology presented itself as a potential research instrument to biologists involved in tissue culture, but film became a powerful tool for researchers because the research program in biology had changed to accommodate it. That is, Braus came to film not only because it solved a particular problem but because the problem itself was shaped by changes in conceptions of growth and time that motion picture technology could articulate (indeed, that the moving image might have prompted). Of course, the practical benefits outlined at the beginning of this chapter provided compelling reasons to use cinematography. In Braus’s case, it offered the opportunity to record his experiments and then to present some of his findings to a wide audience. The photographic or filmic image also projected the impression of objectivity and thereby provided a potent rhetorical weapon in his arguments with the conclusions of other biologists. And film’s ability to manipulate time allowed Braus to present quickly evidence that would have taken hours to observe directly. But beyond these obvious advantages, we could argue that the growth Braus documented was researchable only
because of cinema. The motion picture camera—because it could alter the scale of cellular time through slow-motion or time-lapse cinematography—could capture events that were not otherwise visible. These events were not merely difficult to observe without the camera, in a real sense they did not exist without it. They became, as Walter Benjamin noted, our “optical unconscious,” revealed to us by cinematography in the same way that our psychic unconscious is revealed by—
and comes into being by virtue of—psychoanalysis.
155
But there is more to the success of cinema in science and its relatively rapid appropriation than even these good (practical) reasons. Not only were filmic techniques increasingly available, they were also adaptable to various scientific enterprises. If Seddig focused on the space between the frames, on cinema’s
analytic ability, Braus similarly used the time between exposures to his advantage. But for Braus the image of the object was paramount. The images made little sense analytically; instead, Braus concentrated on their
synthesis. For Braus the gap was crucial for the construction of the film, but the ellipsis was elided in favor of a temporal continuity or biological teleology. Growth is not reversible. This is not to say that film’s analytic abilities were confined to (or more suitable for) the physical sciences, while its synthetic nature was best suited to the life sciences. The variety of applications in a range of disciplines makes any such generalization rash. In fact, as Latour might argue, cinema’s adaptability was no greater or less than that of any technology—from the microscope to the computer. Nor was Braus’s “synthetic” use of cinema any less scientific than Seddig’s “analytic” emphasis. Braus was doing more than “simply” recording a phenomenon and sharing it with others, even though he did not explicitly extract quantitative data from the images. Braus’s use of time-lapse cinematography recorded only certain, regularly paced moments over the continuity of an event, thereby creating, I would argue,
a statistical sampling of the temporal dimension of the phenomenon. We can therefore see Braus’s particular appropriation of motion picture technology as a statistical application of cinematography very much in keeping with the contemporary adoption of statistical thinking for the study of systems in both physics and biology. Indeed, part of the persuasiveness of these records could be found in their “objective,” mathematical construction of a representative sample.
156
Bergson, however, articulated the limits of analysis with respect to dynamic processes, and an extended comparison of the use of motion pictures in physics and biology would expose the paradox of analysis and synthesis as a problem of comprehending continuity through discontinuity. Thinking about Bergson and motion pictures together, in other words, highlights fundamental assumptions in certain scientific approaches about the nature of time and movement. Seddig’s use of motion picture technology to study Brownian motion certainly reveals a commitment to interruption, reversibility, and immobility in the study of dynamic systems—a commitment that was obviously present in Einstein’s equations as well. The ambidexterity of motion picture technology—its dual nature as an instrument of both still and moving images—not only explains its success in science, but its varied and subtle permutations in scientific application can themselves be understood as a form of commentary on scientific theory and method. How researchers used motion picture technology in their experiments tells us much about their disciplinary and philosophical investments.
Above all, I want to stress the mutually reinforcing relationship between science and cinema. The problems and solutions that the technology and the research program presented to each other were entwined and dependent. For Braus and others, motion picture technology fit quite well with other elements of this experimental ensemble, in part because certain formal features—its temporal malleability; its ability to frame and isolate; the forward, teleological motion of its projected image; and so on—seemed to match similar features of the technique of tissue culture. There are other, broader homologies as well. The technique of tissue culture extracted life, reproduced it technologically, and prolonged it, allowing researchers to artificially accelerate or slow life down. And what is cinema except a technology that extracts bodies from their natural time and space, reproducing them mechanically, reanimating them repeatedly (faster or slower as needed) long after the host has expired? The idea that tissues, organs, and life itself are separable from the body—and, by implication, that death is foreign to the organism—is, like cinema, an especially modern notion. So we could say that cinema’s singular facility with time, space, and life made it an ideal instrument to capture and confront these concepts in modern science. But we need not go so far. In fact, such expansive analogies tempt us to think of cinema and science as single entities, when the point of these case studies has been to demonstrate the opposite: that film is not monolithic and that science, like other broad umbrellas, contains many different disciplinary agendas, each of which has adopted some aspects of film technology while ignoring others. That so many different researchers found something to use in motion pictures indeed indicates a special relationship between scientists and film. Braune and Fischer’s deconstruction and reconstruction of the human body, adapting it to modern labor systems; Einstein’s and Seddig’s flexible notions of time expressed in the invisible, random, and reversible world of molecular physics; Harrison’s and Braus’s conceptions of life as separable, temporally malleable, and technologically reproducible—in each case, these scientists turned to motion pictures not merely to observe and record phenomena, but because in cinema they found a kindred spirit, an amiable partner that shared their vision, that could look at the world as they did. The cinematograph, then, was not just a handy tool. In more ways than Bergson imagined, the form and application of motion pictures articulated science’s modern agendas.

In this chapter, we have examined the merger or accommodation of film form and objects, theories, and disciplinary agendas. In each case, film proved its worth because of this correspondence—not exclusively, of course, because the amount of work necessary to adapt film to these aspects of scientific endeavor is prodigious and varied. But we can credit the researchers for recognizing the homology between film and experiment in the first place. The next chapter will examine medical research films and the correspondence between film form and observational practices or ideals.