1 Introduction
Sedimentological studies are of expanding significance in environmental research, especially contamination studies, where past patterns in ecological procedures should be joined with information on current conditions to foresee likely future changes [1]. Sedimentology is a vital branch of geology and its utilization in water resources administration, contamination investigations of subsurface water bodies and depositional condition cannot be over underscored. The presumptions that groundwater is perfect and drilling ought to be done blindly are demonstrations of ignorance and the outcomes far exceed the benefits. Basic sedimentological information should shape some portion of any evaluation of possibly polluted destinations and part of examinations concerning the scattering and catching of contaminants in fluvial frameworks [2]. These information are additionally required for sound ecological administration to guarantee that planning policies are good with common environmental limitations [1].
Sedimentological examination of subsurface borehole sediments is a noteworthy part in groundwater study and aid to predict the depositional condition utilizing fundamental standards of geology [1, 3–5]. The stratigraphy of a landscape assumes an imperative part in groundwater pollution particularly when the aquifer geometry is unconfined [6–8]. When the aquifer is unconfined and shallow, there is a high possibility of contaminants whatever source and kind, particularly when they are near a highly populated area with high anthropogenic effect [9]. Omorogieva and Imasuen [10], Joshua and Oyebanjo [11] estimated that aquifer deposits come from the close surface, igneous outcrops, volcanic and sedimentary rocks. Some of these are effectively disintegrated, though others, particularly the crystalline rocks, are influenced by streams just when adjusted in the surface [12].
It has been perceived that grain-size distribution of granular permeable media influences its hydraulic conductivity [13]. This interrelationship is helpful for the estimation of conductivity properties where coordinate permeability information is inadequate in the beginning times of aquifer investigation. In groundwater hydrology, the learning of soil conductivity is vital for demonstrating the water flow both in the saturated and unsaturated zone, and transportation of water-dissolvable contaminations in the aquifer. The textural properties of an aquifer are all the more effectively acquired, a potential option for evaluating hydraulic conductivity of soils is from grain-distribution [14].
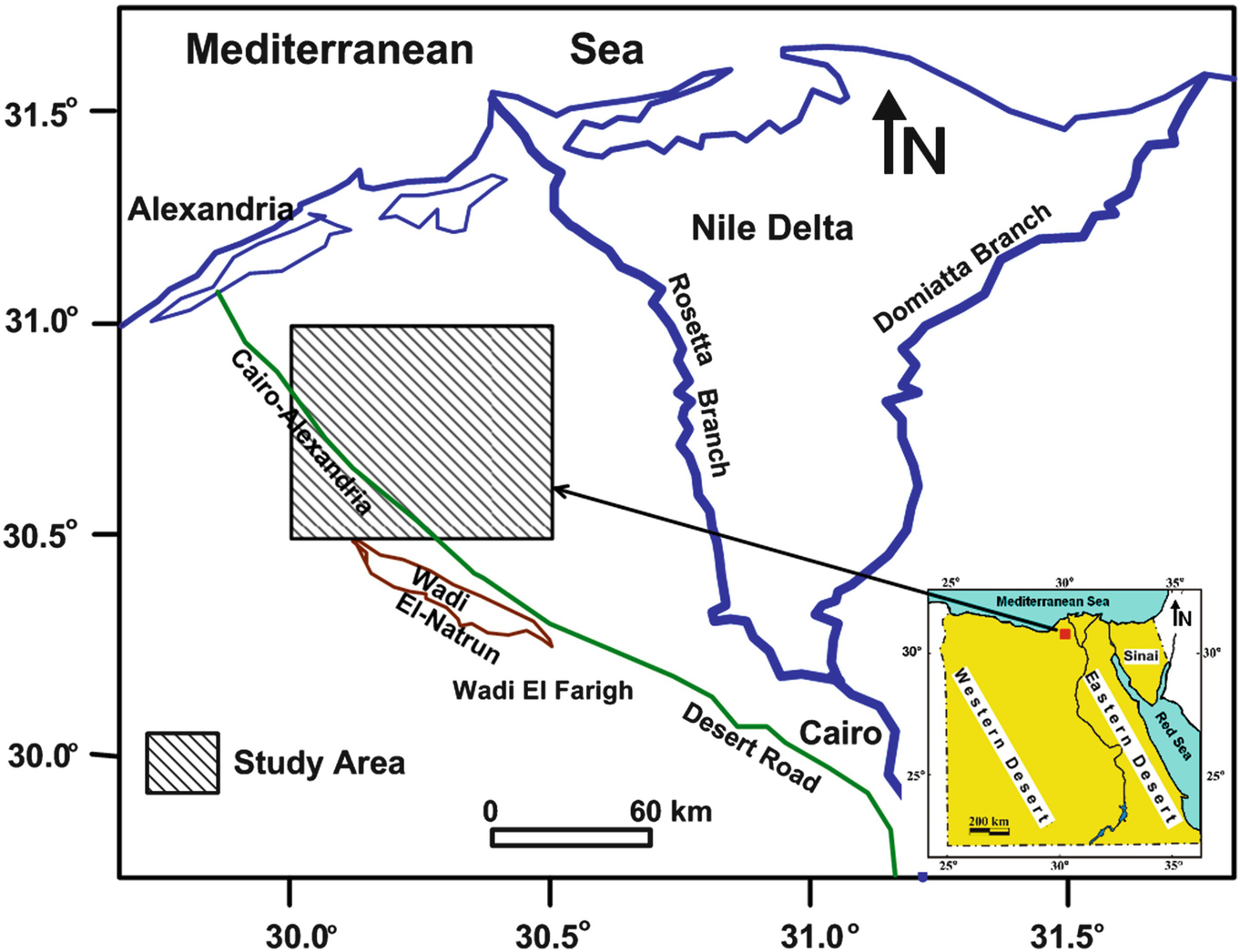
Location map of the study area
2 Study Area
West of the Nile Delta is one of the biggest groundwater territories in northern Egypt. Groundwater potentiality and dispersion are controlled by the geographic location, the sedimentary succession, the geologic structure, and physiographic setting. The study area is situated in the west of the Nile Delta in northern Egypt (Fig. 1). Its geographical boundaries are 30° 30′ and 31° 00′ N and longitudes 30° 00′ and 30° 30′ E. The common territories in this area are north of Wadi El Natrun, El Bustan extension reclamation project, west and east of El Nubaria canal, Abu El Matamir area, and Housh Eissa area.

Shows the hydrogeology of the study area. Pleistocene aquifer thicknesses increase to the eastern and northern directions is shown in (a). The hydro-stratigraphic succession of the study area along the cross-sections AA′ and BB′ is shown in (b) and (c), respectively (after [21])
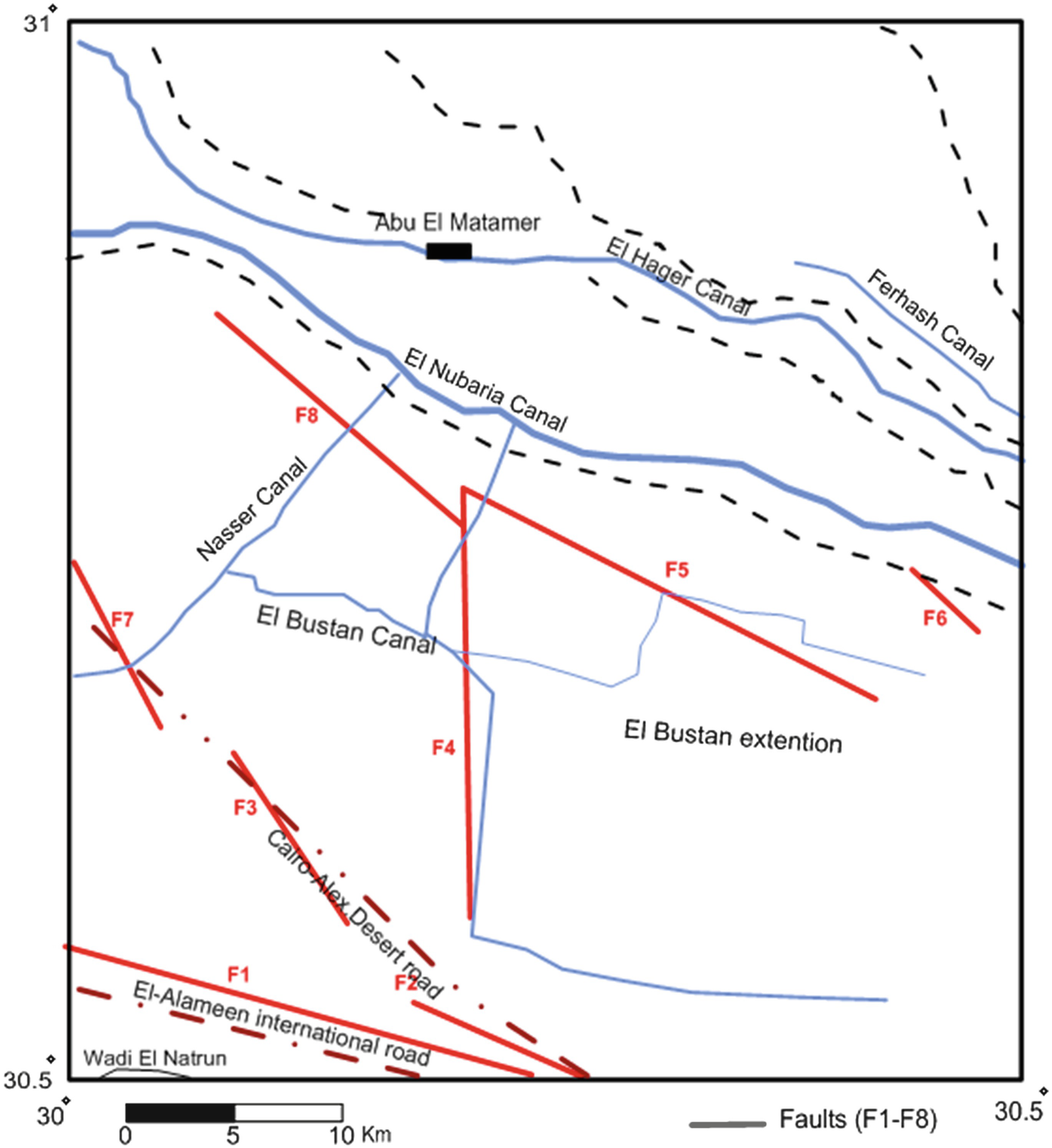
Wadi El Natrun anticlinal structure trends E 35° W and extends for about 60 km from El Ralat depression in the north to Beni Salama depression in the south [20]. It is a symmetrical structure affected by parallel and diagonal faults, among them F1 and F2 that led to the formation of the main central depression (Fig. 3). Unconformities are recognized in the area between the Quaternary deposits and Pliocene deposits, where Wadi El Natrun Formation (Early Pliocene) is covered directly by Quaternary clastics, and El Hagif Formation is absent (Late Pliocene) [18].
3 Methodology

Flowchart shows the working methodology

Borehole and the 2D cross-sections location map

Vertical lithological distribution along the West-East cross-section (AA′, a) and the South-North cross-section (BB′, b)

3D lithology models of the study area
Statistical grain size parameters of the collected samples
Well no. | Sample depth (m) | Md | Mz | SI | SKI | KGI |
---|---|---|---|---|---|---|
1 | 1 | 1.10 | 1.43 | 1.07 | 0.40 | 2.32 |
5 | 0.80 | −0.37 | 3.37 | −0.59 | 1.61 | |
10 | 0.60 | 0.20 | 2.21 | −0.35 | 1.26 | |
15 | 0.50 | 0.33 | 2.21 | −0.31 | 2.00 | |
25 | 0.90 | 0.67 | 1.91 | −0.27 | 1.27 | |
35 | −2.80 | −3.27 | 4.27 | −0.19 | 1.16 | |
2 | 40 | 0.70 | 0.57 | 0.75 | −0.33 | 1.76 |
45 | 1.05 | 1.08 | 0.93 | −0.02 | 1.19 | |
55 | 0.60 | 0.43 | 1.34 | −0.18 | 1.06 | |
60 | 1.70 | 1.67 | 0.83 | −0.14 | 1.69 | |
70 | 0.95 | 0.98 | 0.98 | −0.02 | 1.19 | |
75 | 0.80 | 0.53 | 1.12 | −0.25 | 1.55 | |
90 | 1.30 | 1.13 | 1.07 | −0.35 | 1.45 | |
100 | 0.70 | 0.63 | 1.12 | −0.14 | 1.53 | |
125 | 1.30 | 1.27 | 0.96 | −0.15 | 1.19 | |
140 | 1.40 | 1.33 | 0.90 | −0.16 | 1.12 | |
3 | 20 | 1.10 | 0.93 | 1.31 | −0.37 | 1.64 |
28 | 1.00 | 1.00 | 1.03 | −0.10 | 1.20 | |
48 | 0.90 | 0.77 | 1.27 | −0.24 | 1.39 | |
4 | 20 | 1.30 | 0.90 | 1.24 | −0.42 | 1.07 |
25 | 1.00 | 0.77 | 1.29 | −0.32 | 1.13 | |
30 | 1.30 | 1.03 | 1.14 | −0.40 | 1.23 | |
35 | 1.30 | 1.10 | 1.08 | −0.36 | 1.20 | |
40 | 1.30 | 1.03 | 1.13 | −0.39 | 1.30 | |
50 | 1.00 | 0.73 | 1.40 | −0.30 | 1.18 | |
55 | 0.30 | 0.03 | 1.37 | −0.26 | 1.06 | |
60 | 1.00 | 0.90 | 1.10 | −0.23 | 1.20 | |
65 | 1.30 | 1.03 | 1.13 | −0.39 | 1.20 | |
70 | 1.20 | 0.80 | 1.62 | −0.48 | 1.56 | |
80 | 0.90 | 0.77 | 1.31 | −0.24 | 1.28 | |
90 | 1.50 | 1.43 | 1.11 | −0.17 | 1.08 | |
100 | 0.30 | 0.80 | 1.04 | 0.46 | 1.26 | |
110 | 1.30 | 1.20 | 0.96 | −0.24 | 1.01 | |
130 | 1.50 | 1.33 | 0.99 | −0.25 | 1.16 | |
5 | 1 | −0.70 | −0.87 | 2.07 | −0.07 | 1.23 |
100 | 0.90 | 0.80 | 1.18 | −0.17 | 1.02 | |
110 | 0.80 | 0.67 | 1.36 | −0.17 | 1.13 | |
6 | 10 | 0.90 | 0.57 | 0.94 | −0.51 | 1.23 |
30 | 0.70 | 0.50 | 1.57 | −0.25 | 1.23 | |
50 | −1.00 | −1.80 | 3.78 | −0.38 | 0.86 | |
80 | 0.30 | 0.17 | 0.93 | −0.17 | 1.09 | |
120 | 1.00 | 0.90 | 1.16 | −0.17 | 1.32 | |
150 | 1.00 | 0.97 | 1.01 | −0.04 | 1.10 | |
7 | 27 | 1.00 | −0.30 | 3.33 | −0.72 | 1.43 |
35 | 1.20 | 1.10 | 1.29 | −0.20 | 1.20 | |
43 | 1.00 | 0.93 | 1.08 | −0.13 | 1.11 | |
48 | 0.10 | −0.23 | 1.04 | −0.31 | 1.01 | |
97 | 1.70 | 1.63 | 0.56 | −0.25 | 1.97 | |
106 | 0.80 | 0.67 | 1.26 | −0.14 | 0.84 | |
7 | 120 | 1.20 | 0.93 | 1.25 | −0.29 | 1.26 |
125 | 0.70 | 0.57 | 1.30 | −0.17 | 1.45 | |
8 | 22 | 1.40 | 1.20 | 1.48 | −0.24 | 0.77 |
133 | 1.40 | 1.20 | 1.24 | −0.28 | 0.67 | |
9 | 0 | 1.00 | 1.27 | 1.24 | 0.27 | 0.89 |
40 | 1.00 | 1.40 | 1.28 | 0.25 | 0.98 | |
70 | 1.40 | 1.27 | 0.90 | −0.20 | 1.23 | |
75 | 1.60 | 1.47 | 0.80 | −0.24 | 1.37 | |
80 | 0.90 | 1.03 | 1.07 | 0.00 | 1.27 | |
85 | 1.60 | 1.60 | 1.02 | −0.12 | 1.16 | |
102 | 1.90 | 1.97 | 0.65 | 0.02 | 1.89 | |
11 | 12 | −2.60 | −3.10 | 4.16 | −0.17 | 1.28 |
25 | 1.00 | 0.87 | 1.05 | −0.24 | 1.13 | |
27 | 1.60 | 1.47 | 1.06 | −0.25 | 1.17 | |
30 | 1.00 | 1.10 | 1.01 | 0.09 | 1.10 | |
35 | 1.40 | 1.27 | 0.88 | −0.19 | 1.09 | |
45 | 1.20 | 0.63 | 1.58 | −0.56 | 1.86 | |
55 | −1.00 | −0.97 | 2.28 | −0.12 | 1.04 | |
60 | 0.40 | 0.13 | 1.46 | −0.32 | 1.28 | |
12 | 4 | 1.40 | 1.23 | 1.10 | −0.29 | 1.40 |
13 | 1.60 | 1.50 | 0.86 | −0.14 | 1.19 | |
30 | 0.80 | 0.77 | 1.20 | −0.06 | 1.05 | |
44 | 1.30 | 1.17 | 1.45 | −0.28 | 1.55 | |
13 | 10 | 1.20 | 1.20 | 1.21 | −0.05 | 1.26 |
30 | 1.30 | 1.17 | 1.30 | −0.23 | 1.26 | |
40 | 1.30 | 1.07 | 1.10 | −0.34 | 1.29 | |
50 | 1.10 | 1.13 | 1.53 | −0.21 | 1.52 | |
60 | 1.40 | 1.23 | 1.02 | −0.26 | 1.45 | |
120 | 1.30 | 1.23 | 0.88 | −0.09 | 1.19 | |
135 | 1.00 | 0.83 | 1.29 | −0.19 | 1.13 | |
14 | 4 | 1.30 | 1.20 | 1.83 | −0.16 | 1.17 |
10 | 1.80 | 1.67 | 1.24 | −0.32 | 1.08 | |
30 | 1.6 | 1.47 | 1.11 | −0.28 | 1.37 | |
14 | 85 | 0.80 | 0.37 | 1.81 | −0.42 | 1.64 |
15 | 1.20 | 1.20 | 1.55 | −0.23 | 1.84 | |
15 | 65 | 0.20 | −0.30 | 2.53 | −0.33 | 1.36 |
75 | 1.40 | 1.43 | 1.14 | −0.05 | 1.17 | |
80 | 1.30 | 1.23 | 1.27 | −0.12 | 1.05 | |
85 | 0.80 | 0.90 | 1.23 | 0.11 | 0.96 | |
90 | 1.40 | 1.30 | 1.24 | −0.22 | 1.29 | |
120 | 0.90 | 0.90 | 0.92 | −0.15 | 1.15 | |
130 | 1.20 | 0.90 | 1.47 | −0.43 | 1.64 | |
140 | 0.90 | 1.00 | 1.30 | 0.04 | 1.05 | |
150 | 0.40 | 0.37 | 1.33 | −0.03 | 1.26 | |
155 | 0.20 | −0.03 | 1.51 | −0.27 | 1.07 |

The relationship between mean size (Mz) on one hand and standard deviation (σ1), skewness (SK), and kurtosis (KG) on the other hand of the Pleistocene sediments
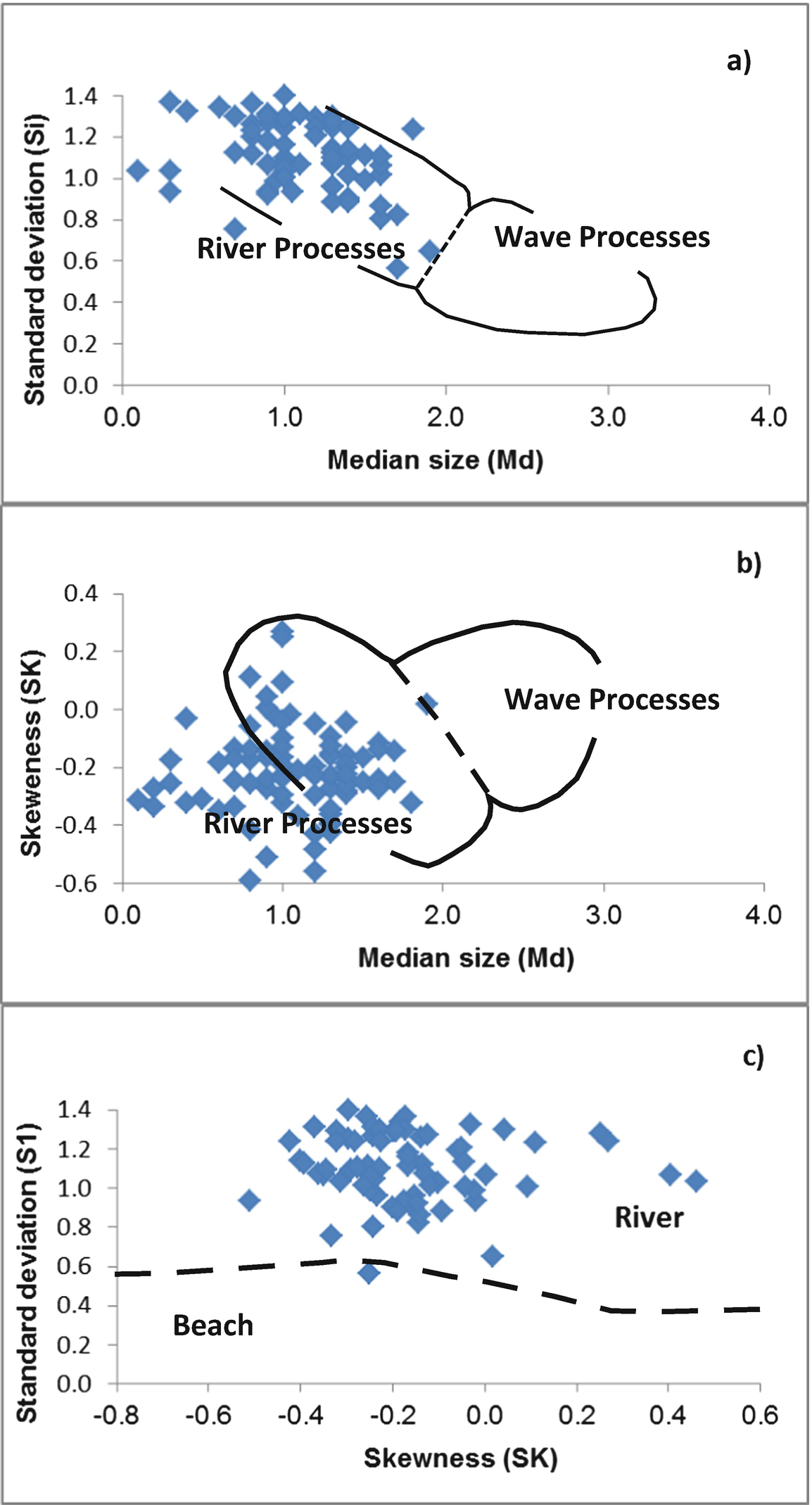
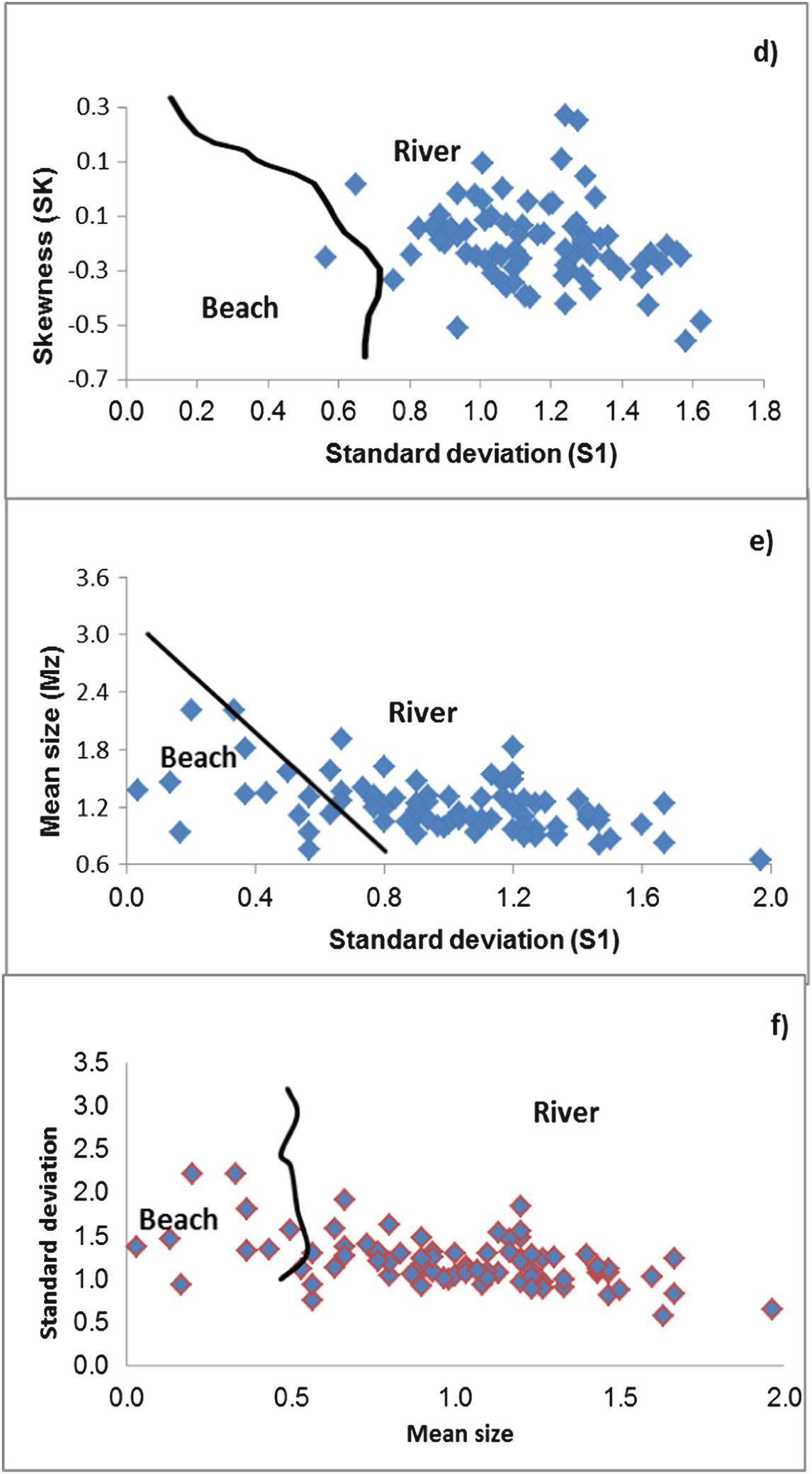

X-Ray Diffractograms of some selected samples in the area. From up to down, these diffractograms are for samples 8 (well 14), 30 (well 9), 36 (well 12), 56 (well 9) and 90 (well 8). Horizontal axis represents two-theta (degree) and the vertical axis represents the relative peak intensity (counts) for each diffractogram
4 Result and Discussion
4.1 Lithological Correlation
The samples from different wells drilled in the area (Fig. 5) are distributed overall the area at various depths (Table 1). The cross-sections AA′ and BB′ (EW and SN, respectively) were established (Fig. 6) to show the vertical and lateral lithology changes. These sections revealed that the sediments of the area are formed of fine to medium sand, gravels, and clay lenses at different levels. Cross-section AA′ (Fig. 6a) extends from E-W direction and contains seven lithology wells [1, 6, 19, 27, 29–31]. The general cross-section composition is sand from fine to coarse size. The sand and gravel sediments which are considered as an ideal groundwater aquifer are recorded in wells 12, 4, 5, and 10. Intercalations of some clay lenses are recorded all over this cross-section. These lenses might affect the level and quality of the groundwater in the investigated aquifer.
Cross-section BB′ contains six wells [23, 27, 32–35] (Fig. 6b) and shows that, the ground level is decreased toward the northern direction. It also indicates that the sand thickness increased to the central part and decreased to the southern and northern directions. In contrast, the number and thickness of the clay lenses increased to the southern and northern directions. Also, the constructed 3D models (Fig. 7) show the lithology variations as represented from AA′ and BB′ cross-sections in all directions. The 3D model shows the increasing of sand thickness toward the central part. Increasing in number and thickness of the clay lenses toward south, north and deeper zones led to decrease in sand thickness.
4.2 Granulometric Analysis
The granulometric study of sediment is an important textural parameter which measures the energy of the depositional environment. Both the friable and semi-friable samples from different wells were selected for the grain size analysis by sieving method. The statistical parameters of Folk and Ward’s [31] were calculated from the grain size data. The data were then plotted on the cumulative curves.
Summary of the grain size parameters of the collected samples
Parameter | Phi value (φ) | Description | |
---|---|---|---|
Mz | Range | −3.27 to 1.97 | Medium pebbles to medium sand |
Average | −0.6 | Very coarse sand | |
Md | Range | −2.8 to 1.9 | Fine pebbles to medium sand |
Average | −0.4 | Very coarse sand | |
σ1 | Range | 0.56–4.27 | Medium well sorted to extreme poorly sorted |
Average | 2.45 | Very poorly sorted | |
SK | Range | −0.72 to 0.46 | Strongly coarse to strongly fine skewed |
Average | −0.13 | Coarse skewed | |
KG | Range | 0.67–2.32 | Platykurtic to Leptokurtic |
Average | 1.5 | Leptokurtic |
4.2.1 Univarient Grain Size Parameters

The mean size value of the Pleistocene samples (Table 2) ranges from −3.27 to 1.97 Φ (medium pebbles to medium sand) with an average value of −0.6 Φ (very coarse sand). The values of mean size fluctuate frequently reflecting the change of the depositing medium.



4.2.2 Bivariate Grain-Size Parameters
The bivariate plots between grain-size parameters are very useful in discriminating the depositional environments of rock [31]. The relationships between the calculated statistical parameters are discussed below, and their bivariate plots are given in Fig. 8.
The scatter plot diagram of the mean size versus the inclusive graphic standard deviation (Fig. 8a) shows that the majority of the samples are scattered around the field of moderately sorted, except some poorly sorted and well sorted samples. It further indicates that the decrease in the mean size of sediment is associated with better sorting.
Skewness has successfully been used by Mason and Folk [35], Friedman [14] and Moiola and Weiser [28] to differentiate between various environments of deposition. Strong skewed samples reflect zones of mixing environments [38]. The plot of skewness versus mean size shows that the majority of the samples are coarsely skewed (Fig. 8b). Such behavior may support the multi-directional depositional currents.
The relationship between mean size and kurtosis (Fig. 8c) further indicates that the present sand is leptokurtic. The leptokurtic character corresponds to what Mason and Folk [35] termed “aeolin flat.” This term does not mean that the sediments were deposited by aeolian agent but means that the present day skin of the sediments is influenced by wind action [30]. This explains the more pronounced leptokurtic characters of the studied samples.
Stewart [25] suggested that the plot of median diameters versus each of standard deviation and skewness is important to differentiate between river and wave processes. The plot of sorting vs. median diameter (Fig. 9a, b) indicates that the total samples lie within the field of river processes. Also, the plot of skewness versus median diameter shows that the total samples lie within the same field.
The plot of skewness versus standard deviation [26] shows the studied samples are distributed in the field of fluvial environments. The total samples belong to the river sands (Fig. 9c, d). The discriminating fields of Friedman [27] show the total samples are distributed within the river processes.
Moiola and Weiser [28] used various combinations of Folk and Ward’s [31] textural parameters for differentiation between different environments. The plot of standard deviation versus mean diameters (Fig. 9e, f) shows that the most samples are distributed within the river processes, while 18% of the total samples lie within the beach environment.
4.3 Mineralogical Analysis
X-ray diffraction data of the collected bulk samples
Well no. | Sample depth | Clay minerals | Non-clay minerals | ||||||
---|---|---|---|---|---|---|---|---|---|
Smectite | Illite | Kaolinite | Quartz | Feldspars | Calcite | Hematite | Dolomite | ||
1 | 45 | 24.0 | 2.1 | 12.5 | 38.5 | 12.5 | 8.3 | 2.1 | 0 |
50 | 5.4 | 1.8 | 8.1 | 12.6 | 4.5 | 63.1 | 4.5 | 0 | |
80 | 23.7 | 7.9 | 11.8 | 28.9 | 9.2 | 13.2 | 5.3 | 0 | |
85 | 17.3 | 5.1 | 7.1 | 45.9 | 9.2 | 10.2 | 5.1 | 0 | |
2 | 6 | 30.6 | 3.3 | 16.5 | 20.7 | 9.9 | 12.4 | 0.0 | 6.6 |
17 | 30.5 | 3.8 | 16.8 | 21.4 | 13.0 | 11.5 | 3.1 | 0 | |
3 | 10 | 34.3 | 3.7 | 13.9 | 23.1 | 11.1 | 9.3 | 4.6 | 0 |
37 | 40.7 | 5.5 | 11.0 | 18.7 | 11.0 | 9.9 | 3.3 | 0 | |
4 | 5 | 5.9 | 5.9 | 16.1 | 16.9 | 5.9 | 47.5 | 1.7 | 0 |
10 | 16.5 | 12.1 | 5.5 | 38.5 | 11.0 | 11.0 | 5.5 | 0 | |
15 | 27.9 | 6.3 | 9.0 | 31.5 | 10.8 | 14.4 | 0.0 | 0 | |
40 | 25.8 | 6.5 | 12.9 | 30.3 | 10.3 | 10.3 | 3.9 | 0 | |
5 | 75 | 33.3 | 0.0 | 16.2 | 18.9 | 14.4 | 14.4 | 2.7 | 0 |
90 | 22.4 | 5.1 | 12.2 | 45.9 | 7.1 | 5.1 | 2.0 | 0 | |
7 | 45 | 15.6 | 6.5 | 9.1 | 37.7 | 15.6 | 10.4 | 5.2 | 0 |
87 | 29.3 | 5.4 | 10.9 | 32.6 | 10.9 | 5.4 | 5.4 | 0 | |
96 | 11.8 | 2.7 | 11.8 | 25.5 | 10.9 | 28.2 | 9.1 | 0 | |
98 | 36.3 | 4.9 | 27.5 | 15.7 | 9.8 | 2.9 | 2.9 | 0 | |
104 | 20.7 | 3.7 | 17.1 | 24.4 | 14.6 | 12.2 | 7.3 | 0 | |
107 | 15.1 | 5.4 | 9.7 | 39.8 | 17.2 | 7.5 | 5.4 | 0 | |
118 | 16.9 | 7.0 | 11.3 | 23.9 | 14.1 | 18.3 | 8.5 | 0 | |
130 | 26.7 | 4.0 | 21.3 | 20.0 | 18.7 | 0.0 | 9.3 | 0 | |
8 | 55 | 32.7 | 5.1 | 22.4 | 25.5 | 0.0 | 10.2 | 4.1 | 0 |
90 | 29.8 | 3.5 | 14.2 | 25.5 | 14.2 | 9.2 | 3.5 | 0 | |
135 | 10.8 | 4.3 | 11.8 | 48.4 | 21.5 | 0.0 | 3.2 | 0 | |
9 | 5 | 29.7 | 5.4 | 12.2 | 27.0 | 10.8 | 9.5 | 5.4 | 0 |
10 | 18.2 | 3.6 | 7.3 | 38.2 | 25.5 | 0.0 | 7.3 | 0 | |
30 | 16.0 | 5.6 | 7.2 | 45.6 | 12.0 | 9.6 | 4.0 | 0 | |
56 | 19.9 | 4.0 | 8.5 | 41.5 | 15.3 | 8.5 | 2.3 | 0 | |
73 | 13.3 | 3.1 | 5.1 | 58.2 | 10.2 | 7.1 | 3.1 | 0 | |
12 | 15 | 38.5 | 3.3 | 13.2 | 24.2 | 9.9 | 11.0 | 0.0 | 0 |
36 | 38.3 | 0.0 | 13.8 | 28.7 | 10.6 | 8.5 | 0.0 | 0 | |
13 | 80 | 23.5 | 5.9 | 17.6 | 36.8 | 16.2 | 0.0 | 0.0 | 0 |
100 | 36.3 | 4.4 | 14.3 | 24.2 | 8.8 | 8.8 | 3.3 | 0 | |
102 | 23.4 | 4.7 | 12.5 | 31.3 | 17.2 | 10.9 | 0.0 | 0 | |
14 | 8 | 41.1 | 4.0 | 11.3 | 17.9 | 7.9 | 14.6 | 3.3 | 0 |
30 | 30.0 | 7.3 | 9.1 | 24.5 | 13.6 | 12.7 | 2.7 | 0 | |
Average | 24.7 | 4.7 | 12.7 | 30 | 12 | 12.1 | 3.8 | 0.2 |
4.3.1 Clay Minerals
Smectite is the most abundant clay mineral in the studied sediments (Fig. 10). Its average value reaches up to 24%. The highest value (41.1%) is recorded in the north-central part of the area at 8 m depth, while the lowest value (5.4%) is obtained in the central part at 50 m depth. Smectite may be formed under authigenic and/or detrital conditions [39, 40]. Authigenic smectite may be formed in both continental and marine environments. Authigenic smectites formed in the continental aqueous sedimentary environment are essentially restricted to saline alkaline lakes and characterize the unusual water chemistries that may develop in these settings [32].
In the marine environment, authigenic smectites may be originated from the alteration of volcanics, mixing of ocean waters and plumes of hydrothermal fluid or halmrolysis (submarine weathering) and direct precipitation on the sea floor [41]. Detrital smectites may be formed under exogenous conditions chiefly upon weathering of basic igneous rocks, in alkaline medium. They also may be formed by secondary detrital degraded lattices (mica or chlorite), where their inter-sheet K+ or Mg++ ions are replaced by water molecules [42].
Kaolinite is the second abundant clay mineral encountered in the studied sediments. The average value of kaolinite reaches 12.6%. The highest value (22.4%) is obtained in the northern part at 55 m depth, while the lowest value (5.1%) at 73 m depth in the central part. Kaolinite usually has a detrital origin, although authigenic kaolinite may also be precipitated from circulating groundwaters that are supersaturated with alumina and silica [33]. Typically, kaolinite develops in continental areas subjected to strong chemical weathering. It usually favors moderately warm to humid and well-drained acidic continental areas, where the rate of precipitation is much higher than the evaporation rate. Under these conditions, chemically soluble elements are preferentially removed leaving residual silica and alumina which are involved in the formation of kaolinite [43]. As kaolinite formation requires acidic leaching conditions, it cannot be formed under marine settings [44].
In the alkaline marine environment, there is no leaching, and the water contains a good deal of dissolved calcium. These environmental conditions favor the formation of smectite rather than kaolinite. A study of weathering processes indicates that kaolinite does not form calcareous parent material until all the carbonate has been removed. This kaolinite is likely to be transported to the marine environment by rivers and/or by the wind. It may also have a diagenetic origin. Velde [45] has shown that kaolinite is generally abundant in the early diagenesis, but as depth and maturity of rocks increase, kaolinite becomes less abundant.
Robert and Kennett [46] linked kaolinite abundances to increased precipitation and deposition during high stand of sea level. The great relief of drainage basins, established during low stand of sea level, favors the formation of kaolinite, especially during warm and humid conditions. However, Robert and Chamley [47] found no significant correlation between the abundances of kaolinite and sea level fluctuations.
Kaolinite is a typical low latitude clay mineral [48] and its concentration increases in tropical regions. Millot [43] assumed that kaolinite is dominant in fluviomarine and lacustrine deposits. Kaolinite formation requires strong leaching environment, an acid reaction medium and the presence of aluminum silicates [40]. The formation of kaolinite requires a favorable climate and an environment of high depleted cations other than Al and Si. Perrin [49] considered kaolinite as the most stable clay mineral that does not undergo appreciable change during transportation. However, diagenetic alteration of kaolinite in the marine environment has been noted by Biscaye [29].
Illite is the less abundant clay mineral encountered in the studied sediments. Its average value reaches up to 24%. Illite has a continental origin according to age determination [34]. It is formed by the weathering or partial hydrolysis of muscovite. Thus, it occurs often in soils derived from the weathering of mica-schist, gneisses, acid and medium igneous rocks in association with smectite and other minerals. Weaver [50] indicated that illite could often be formed from smectite in marine environments.
Diagenetic alteration of montmorillonite and kaolinite into illite generally occurs where sedimentation rates are low and time is also important for the chemical interaction between seawater and clay minerals [51]. Illitization of kaolinite is mentioned by Rumeau and Kulbicki [52]. Potassium and magnesium in seawater would be expected to promote the formation of the more stable clay minerals at the expense of the less stable ones.
4.3.2 Non-clay Minerals
The mineralogical examination has been carried out on some selected carbonate rocks using XRD technique. The data of bulk samples (Table 3) show that the essential carbonate minerals are calcite and rarely dolomite whereas noncarbonate minerals include quartz, feldspar, and hematite. The essential carbonate minerals in the studied samples are calcite and rare dolomite. These minerals are detected by their basal reflection at 3.04A and 2.88A, respectively, and their abundances are calculated by measuring peak height above the background. Calcite is the most common carbonate mineral in the studied samples. It is easily identified by the X-ray diffraction technique where the major peak appears at (3.04A). The average percent of calcite reaches about 11.8%. Dolomite is recorded only in two samples. Its identification is aided by X-ray diffraction technique, where the major peak appears at (2.88A). The average percent of dolomite reaches about 5.9%.
Quartz, feldspar, and hematite are detected in the studied samples. These minerals were detected by their basal reflections at 3.4A, 3.25A, and 2.69A, respectively, and their abundances are calculated by measuring the peak heights above the background. The most common detrital minerals in the samples are quartz and feldspar. Quartz is one of the most common detrital minerals in the samples. It is identified by X-ray diffraction technique where the major peak appears at (3.35A). Table 3 shows that the percentage of quartz ranges from 0 to 62% with a mean value of 10.8%. Feldspar is the second abundant detrital mineral in the samples as its chemical stability is lower than that of quartz. Its mean value is 11.8%. Hematite is the lowest mineral in the studied samples, and it is identified by X-ray diffraction where the major peak appears at (2.69A), its average value is 3.7%.
5 Conclusions
This study gave basic information for the future groundwater dynamics and water–rock interaction research in the study area. The grain size analysis data could help for hydraulic conductivity calculations, and the mineralogical description could answer many questions about the change in groundwater salinity and chemistry along the flow system.
- 1.
The main composition of the aquifer in the area is medium sand to medium pebbles, poorly sorted with clay intercalations.
- 2.
The majority of sediments belong to river sands and river processes with multi-directional depositional currents.
- 3.
The main clay minerals in the intercalated clay lenses of the aquifer are smectite and kaolinite. The essential carbonate minerals include calcite and dolomite whereas noncarbonate minerals are represented by quartz, feldspar, hematite, and gypsum.
6 Recommendations
Lithological, grain size, and mineralogical analysis of the clastic sediments help to explain the water hydrodynamic and salinity behavior in an aquifer. Therefore the result of this work might be used for future groundwater investigation in the study area.
Acknowledgments
The authors are grateful to Tanta University for the financial support offered during the course of this research work. The authors thank the editor Prof. Dr. Abdelazim Negm for his constructive remarks.