Chapter 14
Ions and Voltages
We described in Chapter 2 how membranes are composed of phospholipids arranged so that their hydrophobic tails are directed toward the center of the membrane, while the polar hydrophilic head groups face out. Membranes are a barrier to the movement of many solutes. In particular, small hydrophilic solutes such as ions and sugars cannot pass through membranes easily because, to do so, they would have to lose the cloud of water molecules that forms their hydration shell . Two consequences follow from the fact that membranes are barriers. First, the composition of the liquid on one side of a membrane can be different from the composition of the liquid on the other side. Indeed, by allowing cells to retain proteins, sugars, ATP, and many other solutes, the barrier property of the plasma membrane makes life possible. Table 14.1 shows how five important ions have different concentrations in cytosol and extracellular medium. Second, the cell must make proteins called channels and carriers whose job it is to help hydrophilic solutes across the membrane. This chapter describes how cells make use of this barrier property of membranes.
Table 14.1 Typical Concentrations for Five Important Ions in Mammalian Cytosol and Extracellular Medium
Ion | Cytosol | Extracellular Medium |
Sodium Na+ | 10 mmole liter−1 | 150 mmole liter−1 |
Potassium K+ | 140 mmole liter−1 | 5 mmole liter−1 |
Calcium Ca2+ | 100 nmole liter−1 | 1 mmole liter−1 |
Chloride Cl− | 5 mmole liter−1 | 100 mmole liter−1 |
Hydrogen ions H+ (really H3O+) | 60 nmole liter−1 or pH 7.2 | 40 nmole liter−1 or pH 7.4 |
a Note: The unit n for nano (10−9) is one million times smaller than unit m for milli (10−3).
The Potassium Gradient And The Resting Voltage
Ions are electrically charged. This fact has two consequences for membranes. First, the movement of ions across a membrane will tend to change the voltage across that membrane. If positive ions leave the cytosol, they will leave the cytosol with a negative voltage, and vice versa. Second, a voltage across a membrane will exert a force on all the ions present. If the cytosol has a negative voltage, then positive ions such as sodium and potassium will be attracted in from the extracellular medium. We will begin to address the question of how ions and voltages interact by considering the effect of potassium movements on the voltage across the plasma membrane.
Potassium Channels Make the Plasma Membrane Permeable to Potassium Ions
Potassium channels (Fig. 14.1) are found in the plasma membrane of almost all cells. They are tubes that link the cytosol with the extracellular medium. Potassium ions, which cannot pass through the lipid bilayer of the plasma membrane, pass through potassium channels easily. Other ions cannot go through. The precise shape of the tube, and the position of charged amino acid side chains within the tube, blocks their movement. The channels are selective for potassium.
Figure 14.1 The positively charged potassium ion cannot cross the lipid bilayer, but passes easily through a water-filled tube in a potassium channel.
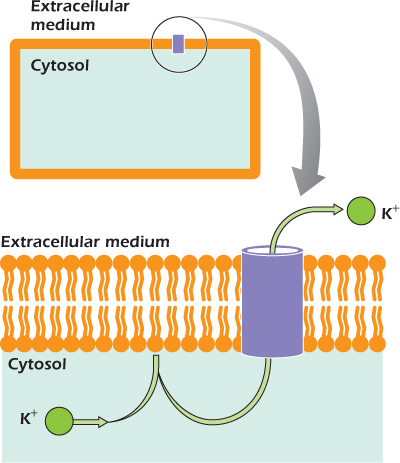
We saw earlier that the sodium/potassium (Na+/K+) ATPase uses the energy of ATP hydrolysis to drive sodium ions out of the cell and, at the same time, bring potassium ions into the cell. This ensures that potassium is much more concentrated in the cytosol than outside—typically 140 mmol liter−1 in the cytosol but only 5 mmol liter−1 in the extracellular medium. There is an apparent paradox here. If potassium can pass through the potassium channel, why is this ion much more concentrated inside the cell than outside? Why doesn't all the potassium rush out? To explain why, we must think about the effects of ion movement on transmembrane voltage.
IN DEPTH 14.1 MEASURING THE TRANSMEMBRANE VOLTAGE
In 1949 Gilbert Ling and Ralph Gerard discovered that when a fine glass micropipette filled with an electrically conducting solution impaled a cell (panel A in the diagram), the plasma membrane sealed to the glass, so that the transmembrane voltage was not discharged. The voltage difference between a wire inserted into the micropipette and an electrode in the extracellular medium could then be measured. By passing current through the micropipette, the transmembrane voltage could be altered.
Twenty-five years later, Erwin Neher and Bert Sakmann showed that the micropipette did not have to impale the cell. If it just touched the cell, a slight suction caused the plasma membrane to seal to the glass (panel B). The technique, called cell-attached patch clamping, can measure currents through the few channels present in the tiny patch of membrane within the pipette.
Stronger suction bursts the membrane within the pipette (panel C). The transmembrane voltage can now be measured. Alternatively, current can be passed through the micropipette to change the transmembrane voltage—this is the whole-cell patch clamp technique. In 1991, Neher and Sakmann received the Nobel prize for medicine.
Concentration Gradients and Electrical Voltage Can Balance
Figure 14.2A shows a glial cell. Many glial cells express only potassium channels in their plasma membranes. For these cells, the cytosol is about −90 mV relative to the extracellular medium. Potassium ions are acted upon by two forces. They would leave the cell under the influence of the concentration gradient, but are pulled in by the negative voltage of the cytosol. For every ion present on both sides of a membrane, it is possible to calculate the transmembrane voltage that will exactly balance the concentration gradient. This voltage is the equilibrium voltage for that ion at that membrane. When cytosolic and extracellular potassium concentrations are 140 mmole liter−1 and 5 mmole liter−1 respectively, the potassium equilibrium voltage at human body temperature is −90 mV. Thus for the glial cell with a membrane voltage of −90 mV, the forces on potassium ions exactly balance and the cell neither gains nor loses potassium. This in turn means that the cytosol is neither gaining nor losing charge, so the membrane voltage does not change. The condition shown, in which the voltage is equal to the equilibrium voltage of potassium, is a stable one. We can see that the condition is stable by thinking about what would happen if the voltage was for some reason perturbed to, for example, −80 mV. At this new voltage the electrical force pulling the potassium ions in is not strong enough to oppose the concentration force favoring potassium loss. Potassium ions would leave and as they did so they would carry their positive charge out, so the cytosol voltage would move in a negative direction. This would continue until the concentration and voltage forces again balance, which would be when the voltage has returned to the potassium equilibrium voltage. In a similar but opposite way, if the membrane voltage were artificially perturbed to a value that is more negative than the potassium equilibrium voltage, potassium ions would move in until the resulting movement of charge has returned the membrane voltage to the potassium equilibrium voltage. In general, a cell whose plasma membrane is permeable to one ion only will have a voltage equal to the equilibrium voltage of that ion.
Figure 14.2 The resting voltage of (A) glial and (B) nerve cells.
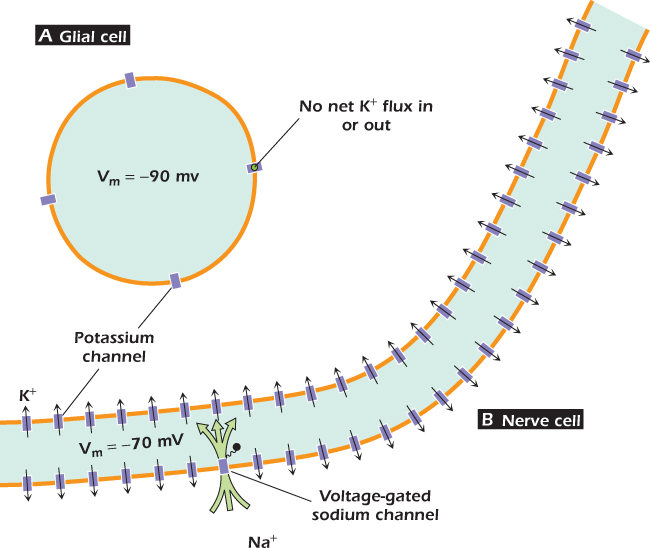
In other cells the situation is more complicated. In nerve cells, for instance (Fig. 14.3), the voltage of an unstimulated cell is −70 mV (Fig. 14.2B). This is because these cells have in addition a second type of channel called the voltage-gated sodium channel that allows sodium ions (and only sodium ions) to pass. As its name suggests, opening of the voltage-gated sodium channel is controlled by the membrane voltage. At −70 mV, the vast majority of voltage-gated sodium channels are shut: only one in every 4,000 are open at any one time. Nevertheless the few channels that are open allow sodium ions to move in, so that in an unstimulated nerve cell the membrane settles down to a steady state voltage in which the inward current through voltage-gated sodium channels is balanced by an outward current of potassium. Potassium flows because the cytosol is no longer negative enough to hold these ions in against their outward concentration gradient. Even in nerve cells, though, the membrane is more permeable to potassium than to any other ion, so the voltage of the unstimulated cell does not deviate very far from the potassium equilibrium voltage. However, in nerve cells and in all other cells in which the plasma membrane has a significant permeability to sodium, the sodium/potassium ATPase has to work constantly to maintain the concentration gradients across the plasma membrane. This is an example of a steady state maintained by the constant expenditure of energy . As we will see, the voltage across the nerve cell membrane changes dramatically when the cell is stimulated and transmits the electrical signals for which it is specialized. The term resting voltage is used for the voltage across the plasma membrane of the unstimulated cell. We also talk about the resting voltage of cells where the membrane voltage never changes, so we say that the resting voltage of a glial cell is −90 mV.
Figure 14.3 A nerve cell from the retina viewed with its nutritive capillaries. Red blood cells are an example of cells whose membrane voltage varies little, while nerve cells are specialized to transmit electrical signals over long distances. Image by Professor David Becker, University College London; used with permission.
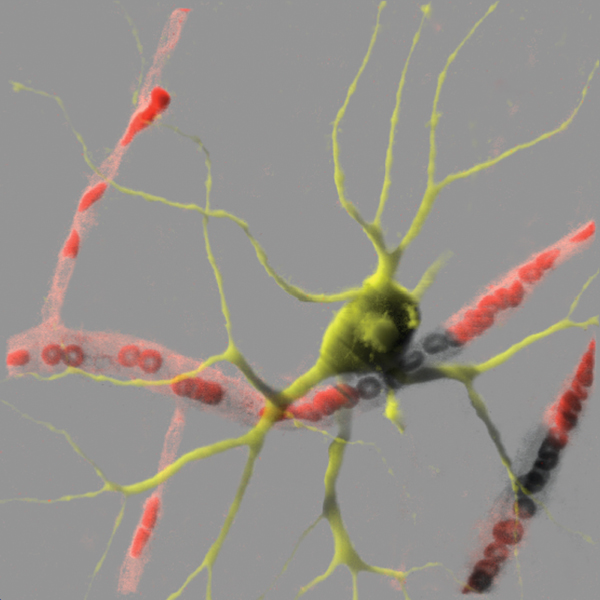
The negative resting voltage characteristic of most cells turns the action of the Na+/K+ ATPase into an energetically asymmetrical one. Consider one conversion cycle: one molecule of ATP is hydrolyzed, three sodium ions are pushed out of the cell, and two potassium ions move in. Very little of the energy of ATP hydrolysis is used up in moving the two potassium ions into the cell because the electrochemical gradient for this ion is close to zero. Although potassium is being moved up a concentration gradient, it is also being pulled in by the negative voltage of the cytosol, and the two forces cancel each other. In contrast, pushing the three sodium ions out of the cell requires more energy than would be required if the cytosol were at the same voltage as the extracellular medium. The sodium ions are positively charged, so they are attracted by the negative voltage inside the cell, which combines with the concentration gradient to form a large inward electrochemical gradient. Most of the energy released by ATP hydrolysis is used to push the three sodium ions up this large electrochemical gradient out of the cell. The presence of the potassium channels, and the resting voltage that they set up, means that almost all the energy of ATP hydrolysis by the Na+/K+ ATPase is stored in the sodium gradient, while potassium ions are close to equilibrium.
Chloride ions (Fig. 14.4) are at a lower concentration in the cytosol than in the extracellular medium. Typically, their concentration in the cytosol is 5 mmol liter−1 compared with 100 mmol liter−1 in the extracellular medium. This is because of the resting voltage set up by the potassium channels. Chloride ions are repelled by the negative voltage of the cytosol. They leave the cell until their tendency to re-enter down their concentration gradient exactly matches their tendency to be repelled by the negative voltage of the cytosol.
Figure 14.4 Chloride is close to equilibrium across most plasma membranes.

IN DEPTH 14.2 THE NERNST EQUATION
An ion that can pass across a membrane is acted on by two forces. The first derives from the concentration gradient. The ion tends to diffuse from a region where it is at high concentration to one where it is at low concentration. The second force derives from the transmembrane voltage. In the case of positively charged ions such as Na+ and K+, the ions tend to move toward a negative voltage. Negatively charged ions such as Cl− tend to move toward a positive voltage. For each ion there is a value of the transmembrane voltage for which these forces balance, and the ion will not move. The ion is said to be at equilibrium, and this value of the transmembrane voltage is called the equilibrium voltage for that ion at that membrane.
When the forces balance, then ions that move in will neither gain nor lose energy. This way of describing equilibrium is useful because it allows us to set equivalent the effects of the two very different gradients, concentration and voltage. For concentration, the free energy possessed by a mole of ions I by virtue of its concentration is
where Go′ is the standard free energy, R is the gas constant (8.3 J mol−1 degree−1), and T is the absolute temperature.
A mole of I passing in therefore moves from a region where it had a free energy of
to one where its free energy is
One mole of ions I moving inward therefore gains by virtue of the concentration gradient free energy equal to
Now consider the electrical force. The definition of a volt means that one coulomb of charge moving across a membrane with a transmembrane voltage of V volts gains V joules of free energy. However, we are working in moles, not coulombs. One mole of ions has a charge of zF coulombs, where z is the charge on the ion. For Na+ and K+ z is 1; for Ca2+ z is 2; and for Cl− z is -1. The term F is a number that relates the coulomb to the mole. It has the value 96,500. Therefore one mole of ions I moving inward gains by virtue of the transmembrane voltage free energy equal to
This does not mean that an ion always gains energy from the transmembrane voltage when it moves inward, as the term zFV can just as easily be negative as positive.
When the effects of concentration and voltage just balance, one mole of ions moving inward neither gains nor loses free energy. Hence, at equilibrium
This can be simplified to
This is the Nernst equation. The value of is 0.025 at a room temperatue of 22°C. At human body temperature
is 0.027.
“In” and “out” can refer to any two solutions separated by a membrane. At the plasma membrane in is the cytosol and out is the extracellular medium, but when considering equilibria across the inner mitochondrial membrane, in is the mitochondrial matrix and out is the intermembrane space.
General Properties Of Channels
Channels are integral membrane proteins that form water-filled tubes through the membrane. We have already introduced three: the connexon , porin , and potassium channels. Channels that, like potassium channels, are selective for particular ions can set up transmembrane voltages. The connexons are much less selective than potassium channels. They form tubes 1.5 nm in diameter through which any solute of Mr < 1000 can pass. Connexons are not always open. They open only when they connect with a second connexon on another cell, forming a tube through which solutes can pass from the cytosol of one cell to the cytosol of the other. Channels that are sometimes open and sometimes shut are said to be gated. When a connexon contacts another on another cell, its gate opens and solute can pass through; at other times the gate is shut. The usefulness of gating is obvious: if the connexons not contacting others were open, many solutes, including ATP and sodium, would leak out into the extracellular medium and exhaust the cell's energy currencies.
Porin in the outer mitochondrial membrane plays an important role in energy conversion. It forms a very large diameter tube that allows all solutes of Mr ≤ 10,000 to pass and seems to spend a large fraction of time open under most circumstances. This is why the outer mitochondrial membrane is permeable to most solutes and ions. Appendix 1 at the end of this book lists all the different types of channels described in this book. It represents only a small fraction of the total number known.
Example 14.1 Cytochrome c—Vital but Deadly
We have described how the electron carrier cytochrome c resides in the intermembrane space between the outer and inner mitochondrial membranes and helps the electron transport chain to convert energy as NADH to energy as the hydrogen ion electrochemical gradient across the mitochondrial inner membrane . Although cytochrome c is a soluble protein of relative molecular mass 12,270, it cannot escape from the intermembrane space into the cytosol because porin, the channel of the outer mitochondrial membrane, only allows solutes of Mr ≤ 10,000 to pass. Although cytochrome c is essential for mitochondrial function, it has another, deadly role. If cytochrome c comes into contact with a class of cytosolic enzymes called caspases, it activates them, turning on the process of cell suicide called apoptosis . Under certain conditions, porin can associate with other proteins to form a channel of larger diameter; when this happens, cytochrome c can leak out and the cell dies by apoptosis. This process seems to occur in hearts during heart attacks, and in the brain during a stroke; therefore a considerable research effort is aimed at preventing this from occurring.
General Properties Of Carriers
We have already met the three carriers that interconvert the four energy currencies of the cell . Carriers are like channels in that they are integral membrane proteins that allow solute to cross the membrane and, like channels, they form a tube across the membrane. However, there is a critical difference. In carriers the tube is never open all the way through; it is always closed at one or other end. Solutes can move into the tube through the open end. When the carrier changes shape so that the end that was closed is now open, the solute can move into the solution on the other side of the membrane.
The Glucose Carrier
One of the simplest carriers is the glucose carrier (Fig. 14.5). It switches freely between a form that is open to the cytosol and a form that is open to the extracellular medium. Inside the tube is a site to which a glucose molecule can bind. On the left, a glucose molecule is entering the tube and binding to the site. Sometimes glucose leaves the binding site before the carrier switches shape. In Figure 14.5 we see the other possibility: the carrier switches shape before the glucose leaves. The binding site is now open to the cytosol, and the glucose can escape into the cytosol. It has been carried across the plasma membrane. Unlike channels, carriers never form open tubes all the way across the membrane. Instead, they bind one or more molecules or ions, then change shape to carry the molecules or ions across the membrane.
The glucose carrier is very simple, whereas other carriers are more complex. We will next consider two carriers, the sodium/calcium exchanger and the calcium ATPase, which do much the same job—they push calcium ions up their concentration gradient out of the cell—but take their energy from different currencies. The sodium/calcium exchanger uses the sodium concentration gradient while the calcium ATPase uses ATP.
The Sodium/Calcium Exchanger
Figure 14.5 shows that the sodium/calcium exchanger, like the glucose carrier, can exist in two shapes, one open to the extracellular medium and one open to the cytosol. Inside the tube are three sites that can bind sodium ions and one site that can bind a calcium ion. The sodium/calcium exchanger is not free to switch between its two shapes at any time. Instead, it only switches if all the sodium sites are filled and the calcium site is empty, or if the calcium site is filled and all the sodium sites are empty.
Figure 14.5 The glucose carrier and the sodium/calcium exchanger are sometimes open to the cytosol and sometimes open to the extracellular medium.
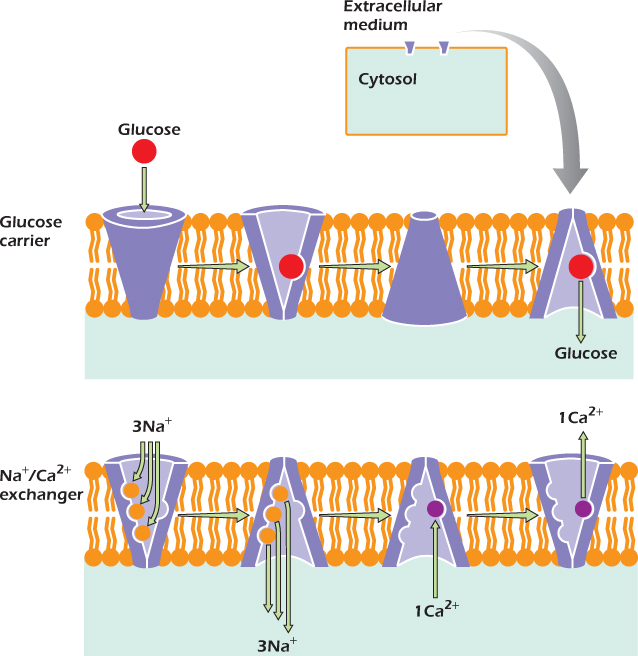
Example 14.2 The Glucose Carrier Is Essential
The cells of our bodies are bathed in a glucose-rich solution. However, glucose cannot cross the plasma membrane by simple diffusion because it is strongly hydrophilic: it can only get in via the glucose carrier. Some cells have glucose carriers in their membranes all the time, but others such as muscle and fat cells translocate the glucose carrier to the plasma membrane only in the presence of insulin. Insulin-dependent diabetics cannot produce their own insulin, and unless they inject synthetic insulin, their muscles and fat cells cannot take up glucose and therefore run out of energy, even though the concentration of glucose in the blood becomes very high. This is why muscular weakness is one symptom of diabetes.
On the left side of the figure, the carrier is open to the extracellular medium. It can switch its shape so that it is open to the cytosol if one of two things happen. It could, as shown, bind three sodium ions (keeping the calcium site empty) or it could bind one calcium and keep the sodium sites vacant. This second option does not often happen, since sodium is at high concentration in the extracellular medium, and one or more of the sodium sites are usually occupied. Therefore nearly all the switches from open-to-outside to open-to-inside are of the type shown. Once the tube has opened to the low-sodium environment of the cytosol, the sodium ions tend to leave.
Once the carrier is open to the cytosol, it can switch back to the open-to-outside form by binding either one calcium or three sodium ions. Since sodium is scarce in the cytosol, the latter event is unlikely. More frequently a calcium ion will bind and will be carried out. The carrier is now ready to bind sodium again and perform another cycle.
The overall effect of one cycle is to carry three sodium ions into the cell down their electrochemical gradient, and one calcium ion out of the cell up its electrochemical gradient. A simple rule about when the carrier can switch shape has produced a machine that uses the energy currency of the sodium gradient to do work in pushing calcium ions out of the cell. Figure 14.6 shows a simple way of representing what is happening. The circle represents one cycle of operation: from open to extracellular medium back to open to extracellular medium again.
Figure 14.6 Action of the sodium/calcium exchanger.
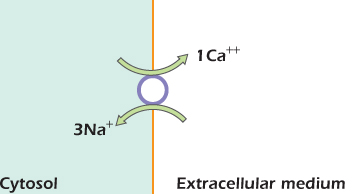
Carriers with an Enzymatic Action: The Calcium ATPase
The electron transport chain, ATP synthase, and the Na+/K+ ATPase are carriers with an additional level of complexity in that they carry out an enzymatic action as well as their carrier function. Figure 14.7 illustrates another carrier with a linked enzymatic function: the calcium (Ca2+) ATPase. Like the sodium/calcium exchanger this is found in the plasma membrane. The transmembrane part of this carrier forms a tube that can be open to the cytosol or to the extracellular medium. Two carboxyl groups in the tube can form a binding site for a calcium ion. Other domains of the protein lie in the cytosol and can hydrolyze ATP. Changes in the shape of the cytosolic region are transmitted to the transmembrane region and force it between the open-to-cytosol and open-to-outside shapes. Figure 14.7 shows our present understanding of how this might happen. (1) is the relaxed shape of the protein. ATP is held by noncovalent interactions in a crevice in one of the cytosolic domains. One of the carboxyl groups in the tube is protonated at this time. (2) A calcium ion moving in from the cytosol pushes the tube open and binds to both carboxyl groups, displacing an H+ ion as it does so. The distortion in protein shape caused by the calcium ion pushing the tube open is transmitted to the cytosolic region and brings the γ phosphate of ATP close to an aspartate residue on a neighboring domain. (3) An intrinsic kinase activity of the two domains transfers the γ phosphate group from ATP to the aspartate residue. This means that a phosphate group, with two negative charges, is sitting very close to ADP, with three negative charges. (4) The repulsion between the phosphorylated aspartate and the ADP forces the two domains apart. The shape change is transmitted to the transmembrane region, which is forced into a wide open-to-outside shape. The calcium ion, which can no longer bind to the carboxyl groups on both sides of the tube, is held only weakly and tends to escape into the extracellular medium. An H+ ion moves in to protonate one of the carboxyl groups. (5) A third cytosolic domain, which has phosphatase catalytic ability, swings in and dephosphorylates the aspartate residue. Meanwhile, ATP moves in and displaces ADP at the binding crevice. Once the aspartate has been dephosphorylated, the protein is no longer held in the open-to-outside shape by electrical repulsion and relaxes back to the resting shape shown in (1). It is now ready to accept another calcium ion from the cytosol and repeat the process. Figure 14.8 summarizes what is happening. The circle represents one cycle of operation. One molecule of ATP is hydrolyzed to ADP and inorganic phosphate, one calcium ion moves out of the cell, and one H+ moves in. The energy released by ATP hydrolysis has been used to drive one calcium ion up its electrochemical gradient and out of the cell.
Figure 14.7 The calcium ATPase undergoes a cycle of phosphorylation and dephosphorylation. These drive shape changes that in turn push calcium ions out of the cell.

Figure 14.8 Action of the calcium ATPase.

Medical Relevance 14.1 Poisoned Hearts Are Stronger
Digitalis is used to treat heart failure. Digitalis inhibits the sodium/potassium ATPase and is extremely toxic. Nevertheless, a small dose, which inhibits the sodium/potassium pump just a little, causes the heart muscle to beat more strongly. The reason is that inhibiting the sodium/potassium pump just a little causes a small increase of cytosolic sodium concentration. Because the sodium/calcium exchanger has three binding sites for sodium, its activity is extremely sensitive to sodium concentration, and even a small increase of cytosolic sodium reduces its activity significantly. The calcium concentration in the cytosol therefore rises. The mechanical motor that drives heart contraction (page 289) is controlled by calcium, so that a small increase of cytosolic calcium makes the heart beat more strongly.
All the cells of our bodies have one of these two calcium pumps, the sodium/calcium exchanger or the calcium ATPase, and many have both. Because of the action of these carriers, the calcium concentration in the cytosol is much less than the concentration in the extracellular medium—usually about 100 nmol liter−1 compared with 1 mmol liter−1. Because the resting voltage is attracting the positively charged calcium ions inward, the overall result is a large electrochemical gradient favoring calcium entry into cells.
For many types of cell the voltage across the plasma membrane never changes. However nerve and muscle cells use changes of transmembrane voltage as a method of rapid signaling over long distances.
The Pain Receptor Nerve Cell
We will illustrate the operation of nerve cells using pain receptors. Figure 14.9 shows the cell responsible for the sensation of pain in a finger. The cell body is close to the spinal cord and extends an axon that branches to the spinal cord and out to the body. The particular pain receptor illustrated in Figure 14.9 sends its axon for almost a meter to the tip of a finger, an extraordinary distance for a single cell. The axon terminal in the skin is the distal, or far away, terminal and the axon terminal in the spinal cord is the proximal one. Potentially damaging events, such as high temperatures, are detected at the finger, and the message is passed on to another nerve cell (a pain relay cell) in the spinal cord. We will now explain how this function is performed.
Figure 14.9 The connections of a pain receptor nerve cell.
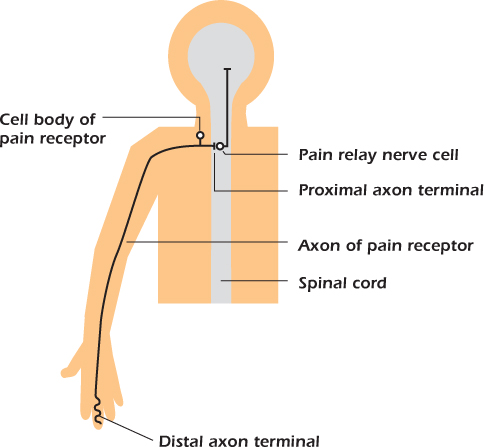
The plasma membrane of the distal terminal contains an ion channel called TRPV that is closed at 37°C but spends a greater and greater fraction of time open at higher temperatures (Fig. 14.10). The channel is a nonselective cation channel, that is, it allows sodium, potassium, and calcium ions to pass. Since even in nerve cells potassium ions are close to equilibrium, the dominant flux through open TRPV channels is an inward movement of sodium and calcium, both of which have a large inward electrochemical gradient.
Figure 14.10 One type of pain receptor has a distal terminal in the skin and is connected to the central nervous system by a myelinated nerve fiber.
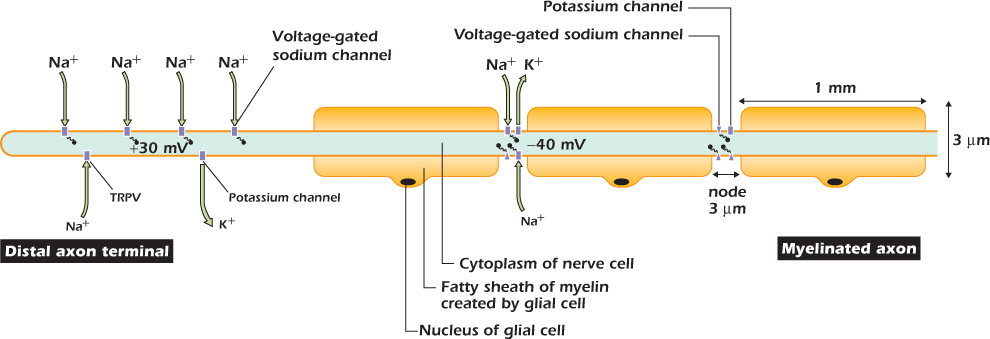
Figure 14.11A shows what happens when the finger is passed through the warm air from a hand dryer. The warmth causes some of the TRPV channels to open, and sodium and calcium ions move in. This inward current causes the transmembrane voltage in the distal axon terminal to depolarize. We use the word depolarization to mean any positive shift in the transmembrane voltage, whatever its size or cause. Because the cytosol is less negative than it was, and hence pulls potassium ions inward less strongly, the outward flux through potassium channels increases. The membrane reaches a new steady state in which the inward current through TRPV channels is balanced by the increased outward current through potassium channels.
Figure 14.11 Electrical events in a pain receptor nerve cell.
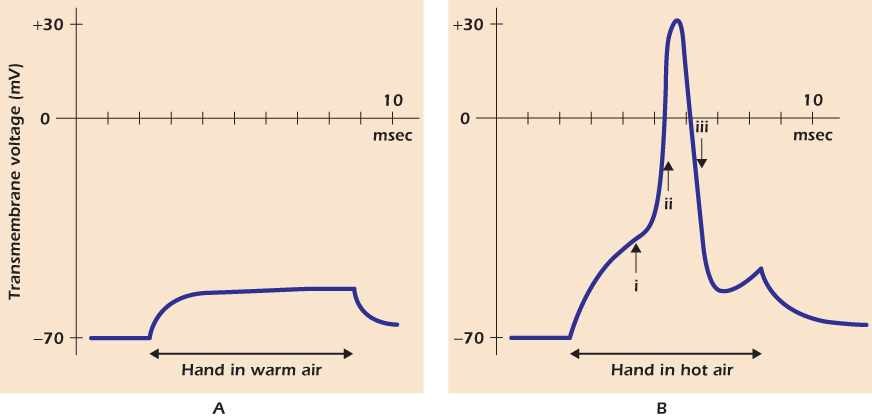
Figure 14.11B shows what happens when the finger is passed through the hot air from a hair dryer. More TRPV channels open, so the distal axon terminal depolarizes further. However, at a voltage of −40 mV, a new phenomenon appears: a massive but temporary depolarization to +30 mV. This is the action potential. Cells capable of generating action potentials are said to be electrically excitable and include nerve cells and muscle cells. To explain the action potential we must return to the voltage-gated sodium channel and describe it in more detail.
The Voltage-Gated Sodium Channel
Figure 14.12 shows how the voltage-gated sodium channel works. On the left is the shape of the protein when the transmembrane voltage is −70 mV. The protein forms a tube, but it is not open all the way through the membrane. Positively charged arginine and lysine side chains are attracted by the negative cytosol, favoring a state in which the tube is gated closed. If the membrane is depolarized, the positive side chains are pulled inwards more weakly and the channel can switch to the open state. Sodium ions can now pass through the channel. A protein domain in the cytosol called the inactivation plug is constantly jiggling about at the end of a flexible link and can bind to the inside of the open channel. The resulting blockage is inactivation, and it occurs after about one millisecond. As long as the plasma membrane remains depolarized, the voltage-gated sodium channel will remain inactivated. When the plasma membrane is repolarized, the positive charges are attracted back toward the inside of the cell, squeezing the plug out.
Figure 14.12 The voltage-gated sodium channel.

In summary:
1. When the transmembrane voltage is −70 mV, the voltage-gated sodium channel is gated shut.
2. When the plasma membrane is depolarized, the channel opens rapidly and inactivates after about 1 ms.
3. After the channel has gone through this cycle, it must spend at least 1 ms with the transmembrane voltage at the resting voltage before it can be opened by a second depolarization.
The voltage-gated sodium channel goes through this process whether or not sodium ions are present. To understand the action potential, we must now think about the effect that movements of sodium ions through this channel have on the transmembrane voltage.
The Sodium Action Potential
Even the slight depolarization in the warm air opened some voltage-gated sodium channels in the distal terminal of the pain receptor, but the additional inward current that they passed was neutralized by the additional outward potassium current, so the membrane voltage stabilized at a new depolarized value. In contrast when the membrane is depolarized past the threshold voltage, which for this nerve cell is about −40 mV, sufficient voltage-gated sodium channels open such that the inward current through them (plus the current through the open TRPV channels) is greater than the outward current through the potassium channels. This is the situation at time (i) in Figure 14.11B. This state is not a stable one because the cytosol is gaining charge and is therefore becoming more positive. More voltage-gated sodium channels therefore pop into the open state, letting more sodium ions pour inward, depolarizing the membrane further. Very rapidly the cell reaches state (ii), where all the voltage-gated sodium channels are open and the membrane is rapidly depolarizing.
There are so many voltage-gated sodium channels in the membrane that the voltage would approach the sodium equilibrium voltage (about +70 mV) but for the fact that the channels inactivate, stopping the sodium influx. By time (iii) almost all the voltage-gated sodium channels are inactivated, so that the only channels that can carry current are the potassium channels. Each of these is now carrying a large outward current since the membrane voltage is much more positive than the potassium equilibrium voltage. The membrane is rapidly repolarizing. Once the transmembrane voltage has returned to negative values, the voltage-gated sodium channels will begin to recover to the closed (but ready-to-open) state, and the cell would soon be ready to generate a second action potential if the TRPV channels were still open.
This figure shows a critical feature of an action potential: it is all or nothing. The warm air did not depolarize the pain receptor sufficiently to evoke an action potential. The hot air depolarized the cell sufficiently to start the process, and the action potential then took off in an explosive, self-amplifying way until all the voltage-gated sodium channels were open and the plasma membrane had depolarized greatly. In all excitable cells there is a threshold for initiating an action potential; in the pain receptor it is about −40 mV. Depolarizations to below the threshold elicit nothing, but depolarizations to voltages more positive than the threshold elicit the complete action potential. At the heart of the action potential is a positive feedback loop. Depolarization causes voltage-gated sodium channels to open, and open sodium channels cause depolarization.
Example 14.3 Chewing off the Inactivation Plug
Proteases are enzymes that hydrolyze the peptide bonds within proteins. In 1976 Emilio Rojas and Bernardo Rudy investigated the effect of introducing protease into squid axons. The membrane of squid axons contains voltage-gated sodium channels that, like ours, normally inactivate about 1 ms after they are opened by a depolarization. However, after introduction of the protease, depolarization caused the voltage-gated sodium channels to open and remain open indefinitely, although the channels would close if the membrane was repolarized. Back in 1976 Rojas and Rudy had no idea why this should be so. Now we can understand the result—the protease cuts the linker between the main part of the voltage-gated sodium channel and the inactivation plug. The plug then floats off and is not available to block the open channel.
The Strength of a Signal Is Coded by Action Potential Frequency
At the distal axon terminal of the pain receptor, the intensity of the stimulus is represented by the amount of depolarization produced. The hotter the air blowing on the skin, the more positive the transmembrane voltage of the terminal becomes. Other sensory cells behave in the same way. For instance, in Chapter 15 we will meet the scent-sensitive nerve cells in the nose that detect smell chemicals. The higher the concentration of the smell chemical, the more these cells depolarize. However, the signal that passes towards the brain is composed of action potentials. The strength of the stimulus cannot be encoded in the amplitude of the action potentials, because they are all-or-nothing discrete events. Rather, the strength of the stimulus is encoded by the frequency of the action potentials.
Figure 14.13 shows how this happens. In Figure 14.13A the stimulus is too weak to elicit an action potential. The graph on the right shows that away from the terminal, the membrane voltage of the pain receptor does not deviate from the resting voltage. Figure 14.13B represents what happens when the stimulus is over threshold, but not by much. After each action potential, the membrane voltage at the distal terminal depolarizes slowly to threshold; the cell therefore fires action potentials at low frequency. The graph on the right shows that the action potentials, but not the TRPV-mediated depolarization, propagate up the axon. Figure 14.13C shows what happens when the stimulus is strong. Because more TRPV channels are open, carrying in positive charge, the depolarization after each action potential is more rapid. Therefore the time between successive action potentials is short and the frequency of action potentials is greater.
Figure 14.13 Frequency coding in the nervous system.

Example 14.4 Peppers and Pain
Capsaicin, the active ingredient in chili peppers, activates one isoform of TRPV channels and so causes the hot, burning sensation in the mouth. In contrast sanshool, the active ingredient in Szechuan peppers, activates pain receptors and other nerve cells by closing potassium channels. This depolarizes the cells to a new steady state voltage. At this new voltage, the outward current of potassium once again has the same amplitude as the inward current through voltage-gated sodium channels. However, since there are fewer open potassium channels, the new steady state voltage must lie further away from the potassium equilibrium voltage such that each potassium channel carries a larger outward current. The depolarization caused is great enough to reach the threshold for action potential generation.
Amplitude modulation (AM) and frequency modulation (FM) are familiar terms from radio. Here we are seeing exactly the same two coding strategies in the nervous system. AM is used in the distal terminals of the pain receptor. The strength of stimulus is coded for by the amplitude of the depolarization. FM is used in almost all axons, including those of the pain receptor. The strength of stimulus is coded for by the frequency of action potentials, each of which has the same amplitude.
Medical Relevance 14.2 A Sodium “Channelopathy”
Epilepsy, in which nerve cells in the brain fire action potentials in an uncontrolled fashion, is not uncommon in young children but often clears up as the child gets older. However, one form of childhood-onset epilepsy, termed GEFS+ for “generalized epilepsy with febrile seizures that extend beyond six years of age,” does not improve with age. Robyn Wallace and coworkers in Australia showed that this condition could be caused by a mutation that slows the rate at which the inactivation plug moves to block the voltage-gated sodium channel. The slow rate of inactivation makes it easier for stimuli to trigger action potentials in the nerve cells in the brain.
Myelination and Rapid Action Potential Transmission
The axon of the pain receptor transmits its signal to the central nervous system so rapidly because for most of its length it is insulated by a fatty sheath called myelin made by glial cells (the glial cells outside the brain and spinal cord are often called Schwann cells). Figure 14.10 shows part of the axon of the pain receptor and its associated glial cells, each of which wraps around the axon to form an electrically insulating sheath. Only at short gaps called nodes is the membrane of the nerve cell exposed to extracellular medium. Note that the vertical and horizontal scales of this diagram are completely different. The axon together with its myelin sheath is only 3 μm across, but the section of axon between nodes is 1 mm long, a large distance by normal cell standards. Between nodes the axon is an insulated electrical cable: just as voltage changes at one end of an insulated metal wire have an almost instantaneous effect on the voltage at the other end, so a change in transmembrane voltage at one node has an almost instantaneous effect at the next node along. The plasma membrane at the nodes has potassium and voltage-gated sodium channels and can therefore generate action potentials.
Figure 14.10 shows the moment at which the action potential is jumping from the axon terminal to the first node. Current has flowed along the axon cytosol from the depolarized terminal to the node, in the same way as current flows down a wire from a point at a positive voltage to a negative one. Current flowing into the node has depolarized it, and the membrane at the node has now reached its threshold for action potential generation. Now, at the node itself, more current is flowing in through voltage-gated sodium channels than is leaving through potassium channels, and the node membrane will rapidly depolarize to about +30 mV. The same thing will now happen along the next millimeter of axon. Current flowing along the axon cytosol will depolarize the next node, and soon it too will generate an action potential. The action potential is jumping from node to node. This process is named after the Latin word meaning “to jump,” saltere, so it is saltatory conduction. For a pain fiber conducting at 15 m s−1 the action potential takes 67 μs to jump each 1 mm distance. The fastest axons in our bodies conduct at 60 m s−1, with each node-to-node jump taking only 17 μs.
TRPV channels are found only in pain receptor nerve cells. Other sensory neurons are depolarized by other stimuli such as cold or touch, while the nerve cells that send commands out from the brain, or process information within it, are triggered to fire action potentials by other mechanisms that we will describe in the next chapter. However all these cells express voltage-gated sodium channels and so all share the mechanism of action potential propagation that we have described.
Example 14.5 Local Anesthetic, Overall Well-being
Nerve conduction is exploited when a dentist uses local anesthetic. A patient feels pain from the drill because pain receptors in the tooth are depolarized and transmit action potentials toward the brain. The site at which they are being depolarized is inside the tooth and therefore inaccessible to drugs until the drill has made a hole. However, the axons of the pain receptors run through the gum. Local anesthetics injected into the gum close to the axon bind to the nerve cell membrane at the node and prevent the opening of voltage-gated sodium channels. Drilling into the tooth still depolarizes the pain receptor membrane, and action potentials begin their journey toward the brain, but they cannot pass the injection site because the nodes there cannot generate an action potential. The patient therefore feels no pain.
The combination of axon plus myelin sheath is a nerve fiber. The nerve fiber shown in Figure 14.10 has an overall diameter of 3 μm. The human body contains myelinated nerve fibers with diameters ranging from 1 μm, conducting at 6 m s−1, to 10 μm, conducting at 60 m s−1. Some nerve cells have axons that are not myelinated and generally conduct more slowly than myelinated fibers. The axons of scent-sensitive nerve cells are an example. Getting scent information from the nose to the brain as fast as possible is apparently not a significant advantage, given that a scent can take many seconds to waft from its source to our nose.
Medical Relevance 14.3 Solute Movement in the Kidney
We give here a brief introduction to some of the many channels and carriers, and many complex control mechanisms, that operate in the kidney.
The operational unit of the kidney is the kidney tubule, shown in the upper part of the diagram. The liquid in the tubule is formed by filtering blood and initially has a composition similar to blood plasma. Solutes and water are then added and removed as the liquid passes down the tubule to be finally lost as urine.
One of the functions of the proximal convoluted tubule is to recover the glucose from the tubule fluid. The lower part of the diagram shows how this is achieved. The plasma membrane facing the interstitial fluid (this is called the serosal membrane) contains the glucose carrier we have met before . This will always carry glucose from areas where it is at high concentration to areas where the concentration is lower. In contrast the plasma membrane facing the tubule lumen (this is the luminal membrane) contains an unusual glucose carrier which carries sodium and glucose in. The two movements are locked together: if sodium moves, glucose moves, and vice versa. Sodium is at a low concentration in the cytosol due to the action of the sodium/potassium ATPase . The sodium, glucose cotransporter allows sodium to move down its electrochemical gradient into the cell and at the same time pulls glucose into the cells up its concentration gradient. The result of the glucose inward pumping is that the glucose concentration in the cytosol of kidney tubule cells is much greater than in normal cells (In Depth 12.3 on ) at about 40 mmole liter−1. The glucose can then flow down its concentration gradient, using the regular glucose carrier in the serosal membrane, into the interstitial fluid and hence back into the blood.
Carriers have a maximum rate at which they can work—the relationship between flow rate and concentration follows the same Michaelis-Menten relationship that governs enzymes . The carriers in the kidney can recover almost all the glucose if the concentration in the initially filtered plasma is in the normal 3–4 mmole liter−1 range, but if it increases above 12 mmole liter−1, as happens in the disease diabetes mellitus, then even working at maximum rate the carriers cannot recapture all the glucose and some is lost in the urine, which is therefore sweet, giving the disease its name.
Another function of the kidney is to excrete H+. Metabolism of the sulfur-containing amino acids methionine and cysteine acquired in the diet creates sulfuric acid, which ionizes to sulfate and H+. The diagram shows the processes in the distal convoluted tubule by which the H+ is excreted. At the heart of the process is the H+ ATPase which pushes H+ into the lumen. This carrier is a close relative of mitochondrial ATP synthase (In Depth 12.2 on ). Like ATP synthase it uses a rotor system to couple the ATP ↔ ADP + Pi reaction to the movement of H+ across the membrane. However its job is the reverse one, to hydrolyse ATP and use the energy hydrolysis to drive H+ up its electrochemical gradient.
The energetics of the ATPase are finely balanced, so that even a small fall in the energy released upon ATP hydrolysis will mean that it cannot push H+ out of the cells. Thus any condition that reduces [ATP] or increases either [ADP] or [Pi] in kidney cells will tend to cause acid excretion to fail, leading to a dangerous increase of blood acidity. This condition, called renal tubular acidosis, can happen if the oxygen supply to the kidney is compromised, for example in sickle cell anemia . It can also be simply a consequence of kidney cell stress during the body's response to any disease.
When the adrenal gland secretes the hormone aldosterone, distal convoluted tubule cells express the ENaC sodium channel (Medical Relevance 6.2 on ). The result is that sodium that would otherwise be lost in the urine is recovered. The diagram shows how this is achieved. The luminal membrane already contains channels selective for potassium ions, called ROMK. When ENaC appears, sodium moves in down its electrochemical gradient, because unlike the voltage-gated sodium channel , ENaC spends about 30% of its time open, whatever the membrane voltage. Because this membrane now has a significant permeability to sodium, the voltage across it deviates significantly from the potassium equilibrium voltage; the value moves to about −70 mV. Potassium ions therefore move from the cells into the lumen.
The sodium ions are now pumped out of the cell into the interstitial fluid by sodium/potassium ATPases located on the serosal membrane. This brings potassium into the cells. The serosal membrane is highly permeable to potassium because of the presence of a second type of potassium channel called Kir. However, the serosal membrane is not particularly permeable to other ions, so this membrane voltage has a value close to the potassium equilibrium voltage. There is therefore almost no electrochemical gradient for potassium at this membrane. Rather, the potassium that enters the cells in the sodium/potassium ATPase leaves via ROMK into the lumen.
Patients whose kidneys are excreting too much potassium and resorbing too much sodium are often given the drug amiloride (sold as Midamor), which blocks ENaC. With ENaC blocked, sodium can no longer move into the cells from the lumen and is instead lost in the urine. The luminal membrane is now permeable almost exclusively to potassium, so that the transmembrane voltage across the membrane facing the lumen shifts to a value close to the potassium equilibrium voltage and potassium stops moving out from the cells into the lumen.
Patients with a genetic lesion in ROMK lose excessive amounts of sodium in their urine (Example 9.1 on ). However, a similar syndrome is seen in genetically normal individuals whose body tissues become too acid (acidosis). The primary cause of the acidosis can be a respiratory problem that prevents the patient from breathing out all the carbon dioxide that their cells make, or can be metabolic, as in diabetic acidosis (Medical Relevance 2.1 on ). Whatever the primary cause, a relatively slight acidification of kidney cells markedly reduces the time ROMK spends open. The molecular basis of this phenomenon is still under investigation, but part of the explanation lies in a series of four histidine residues at the carboxy terminus of each of the four identical protein subunits that make up the working channel. The histidine side chain has a pKa of 7 so that an increasing fraction of the sixteen histidines in the complete channel complex are protonated, and therefore carry a positive charge, as the pH falls. These charges disrupt the quaternary structure of the complex and the channel closes so that potassium cannot move from the kidney cells into the tubule lumen. The result is that acidotic patients usually also have a high potassium concentration in their blood plasma (hyperkalemia). If untreated the effects of the high potassium on the heart in particular can be rapidly lethal.
1. Channels are membrane proteins with a central water-filled hole through which hydrophilic solutes, including ions, can pass from one side of the membrane to the other. Changes in protein structure may act to gate the channel but are not required for movement of the solute from one side to the other.
2. The presence of potassium channels in the plasma membrane, and the resting voltage that they set up, means that almost all the energy of ATP hydrolysis by the Na+/K+ ATPase is stored in the sodium gradient, while potassium ions are close to equilibrium.
3. The resting voltage repels chloride ions from the cell interior.
4. Carriers, like channels, form a tube across the membrane, but the tube is always closed at one end. Solutes can move into the tube through the open end. When the carrier changes shape, so that the end that was closed is open, the solute can leave to the solution on the other side of the membrane.
5. The glucose carrier is present in the plasma membrane of all human cells.
6. The sodium/calcium exchanger is a carrier that uses the energy of the sodium gradient to push calcium ions out of the cell.
7. The electron transport chain, ATP synthase, the sodium/potassium ATPase, and the calcium ATPase are both carriers and enzymes, the two actions being tightly linked.
8. The voltage-gated sodium channel is shut at the resting voltage but opens upon depolarization. After about 1 millisecond the channel inactivates.
9. While the voltage gated sodium channel is open, sodium ions pour into the cell down their electrochemical gradient. The mutual effect of current through the channel on transmembrane voltage, and of transmembrane voltage on current through the channel, constitutes a positive feedback system. If the membrane is initially depolarized to threshold, the positive feedback of the sodium current system ensures that depolarization continues in an all-or-nothing fashion. Repolarization occurs when the voltage-gated sodium channel inactivates. The entire cycle of depolarization and repolarization is called an action potential.
10. Action potentials are all-or-nothing events. The strength of the signal transmitted by a nerve cell is encoded not by the amplitude of the action potentials but by their frequency.
11. Long nerve cell processes called axons transmit action potentials at speeds up to 60 m per second. They can transmit the signal at such high rates because myelin, a fatty sheath, insulates the 1 mm distances between nodes.
12. A number of axons are not myelinated; these generally conduct action potentials more slowly than do myelinated axons and are found where high conduction speed is not required.
REVIEW QUESTIONS
14.1 Theme: Cytosolic and Extracellular Concentrations of Important Ions
A. ≥ 10−1moles liter−1
B. 5 × 10−2 moles liter−1
C. 10−2 moles liter−1
D. 5 × 10−3 moles liter−1
E. 10−3 moles liter−1
F. 10−4 moles liter−1
G. 10−5 moles liter−1
H. 10−6 moles liter−1
I. ≤ 10−7moles liter−1
From the above list of concentrations, choose the one that most closely approximates the concentration described in the statements below. Answer for a general human cell.
1. cytosolic calcium
2. extracellular calcium
3. cytosolic chloride
4. extracellular chloride
5. cytosolic H+ (H3O+)
6. extracellular H+ (H3O+)
7. cytosolic potassium
8. extracellular potassium
9. cytosolic sodium
10. extracellular sodium
14.2 Theme: Pathways for Solute Movement Across the Plasma Membrane
A. calcium ATPase
B. connexon
C. glucose carrier
D. potassium channel
E. sodium/calcium exchanger
F. TRPV
G. voltage-gated sodium channel
From the above list of channels and carriers, select the protein corresponding to each of the descriptions below.
1. An enzyme that carries out a hydrolytic reaction
2. A protein with no enzymic activity that carries one solute in one direction and a second solute in the opposite direction
3. A channel that can pass glucose
4. A protein whose expression in almost all human cells is responsible for the fact that their cytosol is at a negative voltage with respect to the extracellular medium
5. A channel expressed in pain receptor neurons which opens at damagingly high temperatures
6. A channel expressed in almost all neurons, even those that are not sensitive to painful stimuli, but which is not expressed in most non-neuronal human cells (it is not found, for example, in liver or blood cells)
14.3 Theme: Ion Fluxes in a Nerve Cell
A. Cl−
B. H+ (H3O+)
C. K+
D. Na+
From the above list of ions, select the ion corresponding to each of the descriptions below.
1. Although gradients of this ion play a crucial role at other cell membranes, the concentration gradient of this ion across the plasma membrane is small: the concentration in the cytosol is the same, within a factor of two, as the concentration in the extracellular medium.
2. In a resting nerve cell, this ion is constantly leaking into the cell, and must be removed by the action of an ATP-consuming carrier.
3. In a resting nerve cell, this ion is constantly leaking out of the cell, and must be pumped back in by the action of an ATP-consuming carrier.
4. The more a nerve cell is depolarized, the greater the electrochemical gradient favoring entry of this ion into the cell.
5. In a resting nerve cell, this ion is at equilibrium across the plasma membrane.
THOUGHT QUESTION
In kidney failure, the concentration of potassium in the extracellular medium can rise from its normal value of 5 mM to 10 mM or even higher. What will be the effect of this on the resting voltage of heart muscle cells? What will be the consequent effect on voltage-gated sodium channels in the heart?
Ashcroft, F. M. (2000) Ion Channels and Disease, Academic Press, San Diego, CA.
Levitan, I. B., and Kaczmarek, L. K. (2002) The Neuron, 3rd edition, Oxford University Press, New York.