Introduction
Chalcopyrite (CuFeS2) is the most important mineral for production of metallic copper and its dissolution behavior represents the most important technological consideration in the hydrometallurgical extraction of copper . Hydrometallurgical copper extraction for low-grade chalcopyrite ores has been attracted much attention from many perspectives such as the ability to deal with a large spectrum of impurities, applicability to low-grade materials, and feasibility of low-capacity operation. Many lixiviants such as sulfates, chlorides and ammonia have been reported for copper leaching . Due to the slow leaching kinetics for chalcopyrite , there are many investigations on the mechanism of the oxidative dissolution of this mineral in order to understand the reason for slow dissolution rate . Although it is widely accepted that dissolution of chalcopyrite is retarded by the inhibition surface layers [1, 2], the surface chemistry during leaching has not been well understood till now. However, despite the enhanced understanding today, no universal agreement has been reached on the composition and microstructure of the inhibition layers, and the role of the layers during the leaching process.
The inhibition layer is less reactive than chalcopyrite , furthermore the compact layers form a barrier to lixiviant access by chalcopyrite , and thereby hinder dissolution. It can be concluded from the literature [3] that the composition of the passivation layers, which are the intermediate products, are dependent strongly on the anodic potential of the reactive chalcopyrite . A number of surface species in the layers have been proposed for the ammonia leaching system, such as Fe2O3 [4], iron -based precipitation layer [5] and Cu1−xFeS2 [6]. Despite some researches about the composition and morphology of the inhibition layer on the leached chalcopyrite [7], there is no study found about the correlation between morphology of the inhibition layers and the anodic potentials of the chalcopyrite . Since the variously anodic potentials on chalcopyrite can simulate the functions of different oxidants used in the oxidative leaching of chalcopyrite , this correlation is important to more completely understand the morphology of the inhibition layers and its relationship with the mechanism of passivation .
In this study, based on the voltammetry measurements, the dependence of composition and morphology of the passivation layers on the chalcopyrite with anodic polarization is presented in combination with XPS analyses and metallographic microscopy. Furthermore, the in-depth compositional profiles of the layers on the oxidized chalcopyrite were evaluated with XPS depth profiling. This information is critical to understanding the surface chemistry during chalcopyrite leaching .
Experimental
High -purity polycrystalline chalcopyrite samples were obtained from GuizhouProvince, China, and there was no impurity phases detected for the samples by XRD analysis. The samples (∅ 3 × 10 mm) were fabricated into cylindrical electrodes, mounted in epoxy resin holders, connected with copper wire using silver epoxy and polished with 2.5 µm diamond abrasive. All electrochemical measurements were driven by a BioLogicHCP-803 High Current Potentiostat, in a three-electrode system with the saturated calomel electrode (SCE) serving as a reference electrode, a graphite plate as a counter electrode, and a chalcopyrite electrode as the stationary working electrode. The electrolyte solution contained 1 M NH4OH and 2 M NH4Cl (pH ~ 8.5) at 35 °C, and was no agitated and left to the open atmosphere.
After the potentiostatic polarization the samples were rinsed with deionized water, dried in air, and characterized with optical microscopy (OM, DM4000 M, Leica), scanning electron microscope (SEM , SU-1500, HITACHI), and X-ray photoelectron spectroscopy (XPS , Fisher Scientific, ESCALAB). Some samples presented in Fig. 3 were collected by adhesive tape to separating the layers from the bulk chalcopyrite . The in-depth composition analysis of the cubic sample, which was leached in the electrolyte solution at OCP, was carried out by alternating XPS measurements and Ar+ sputtering. The etching depth was detected from the profilometer (Dekta 840, Veecog) analyses, and an etching rate was about 0.1282 nm/s.
Results and Discussion

Linear sweep voltammograms with scanning rate of 20 mV/s in 1 M NH4OH and 2 M NH4Cl solution, solid line for the chalcopyrite electrode, dash line for platinum electrode. The inset figure (a) is the open circuit potential-time curve for the chalcopyrite electrode, and inset (b) is the assembly of chalcopyrite electrode

![$$ 3[{\text{O}}_{ 2} ] {\text{ + 6H}}_{ 2} {\text{O + 12}}e^{ - } {\text{ = 12OH}}^{ - } $$](../images/468727_1_En_127_Chapter/468727_1_En_127_Chapter_TeX_Equ2.png)
On the Pt electrode, the anodic current increased rapidly above 0.8 V due to oxygen evolution. The anodic features of the chalcopyrite electrode were illustrated between the rest potential and 0.7 V, which could be divided into two main potential regions. At potentials below 0.3 V, the anodic current density was very small and increased slowly. As the potential increased above 0.4 V, the current increased rapidly with the applied potential, and metal-depleted sulfide should be reduced and decomposed.

a Optical microscopy photographs of the surface with leaching from 0 to 250 h under 1 M NH4OH and 2 M NH4Cl solution at 35 °C. b XPS depth (shown as sputtering time analysis of the sample leached for 100 h (shown as sputtering time with an estimated etch rate of 0.1282 nm/s)
Figure 2b shows XPS spectra measured for the 100-h leaching sample surface (Fig. 2) after sputtering the surface at different depths. The appearance of Fe–O (711.4 eV), O2− and –OH (530.0, 531.4 eV) from the curves of Fe 2p and O 1s suggests that FeOOH is contained on the top layer. The atomic ratio of OH− to O2− decreased from 1.3 to 0.6 corresponding to the initial surface and the surface that was sputtered for 400 s. It can be deduced that the distribution of FeOOH and Fe2O3 with the depth was inhomogeneous, and the composition of FeOOH reached a maximum at the top surface. The Fe–O was totally removed and substituted by Fe–S after the third sputter cycle according to the Fe 2p and O 1 s spectra. The surface layer containing of FeOOH and Fe2O3 should be thinner than 180 nm based on an estimated etch rate of 0.1282 nm/s and a time of 1400 s. There were no sulfur and copper signals detected from S 2p and Cu 2p spectra on the top surface although the blue color of the sample was ascribed to covellite. However, the spectra of Cu 2p and Fe 2p showed that some Fe atoms and almost all Cu atoms were associated with sulfur after the first sputter cycle. The S 2p spectra presented in Fig. 2b consisted of two major doublets representing monosulfide and disulfide. The presence of S22− on the surface after the first sputter cycle suggests iron atoms were removed from the chalcopyrite and formed the oxide, then S2− was oxidized to S22−.

Potentiostatic polarization of chalcopyrite electrode with the corresponding optical microscopy photographs under 1 M NH4OH and 2 M NH4Cl solution
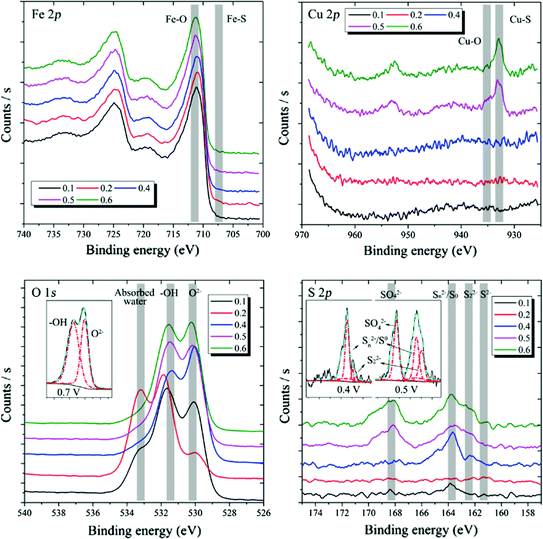
Comparison of Fe 2p, Cu 2p, O 1s and S 2p peaks of the layers separated from the surface of chalcopyrite electrode under the different polarizations for 5 h

a Optical microscope photograph of electrode surface at the anodic potential of 0.4 V for different times and b SEM image of the sample for 45 s
Conclusions
Oxidation reactions on the chalcopyrite electrode at the OCP occurred in the electrolyte solution of 1 M NH4OH and 2 M NH4Cl at 35 °C. The distribution of FeOOH and Fe2O3 with the depth in the oxidized layer was inhomogeneous, and there was accumulation of FeOOH at the top surface based on the XPS results. When the anodic potentials were lower than 0.3 V, the anodic polarization had little effect on the composition and morphology of the oxidized layers formed due to the oxidation of the chalcopyrite electrode compared with those of the chalcopyrite at the OCP. When increased up to 0.4 V, and even higher to 0.7 V, the rate of chalcopyrite oxidation increased dramatically, and the product layers with cracks were fragile and easily exfoliated from the bulk chalcopyrite . The sulfide can be oxidized to sulfur species with higher oxidation states. Furthermore, the oxidation of the chalcopyrite seemed to undergo a cyclically oxidative process that results in exfoliation of the oxide layer that exposes the bulk chalcopyrite in a layer-by-layer sequence.
Acknowledgements
The authors acknowledge the National Program on Key Basic Research Projects of China (Grant No. 2014CB643405) and the Independent Research and Development Project of State Key Laboratory of Pressure Hydrometallurgical Technology of Associated Nonferrous Metal Resources (Grant No. yy2016009).