Introduction
The current dominant REE separation technology, solvent extraction , is more than 50 years old and can be assumed as a huge chemical plant (solvent intensive). The objective of this project is to develop a sustainable alternative REE separation process by exploiting variances in other properties of REE rather than that employed in solvent extraction .
Previously, the author demonstrated continuous separation of rare earth ions based on electrophoretic migration across a traverse laminar flow of a complexing agent , so-called free flow electrophoresis (FFE) [1]. Although FFE separation of REEs has been successfully exercised in the laboratory, scaling the technology to operate in a high throughput refinery plant has been considered to be the major technical challenge. There are several key factors which would affect the scale-up adversely. For instance, FFE devices and the corresponding separation mechanism depend on the development of laminar flow, of background electrolyte (BGE), between two plates spaced several millimeters apart. Although other dimensions of the separation cell have no theoretical limit, the plate spacing is considered a major limitation to the design of high throughput separation system. Uniform flow distribution in between plenty of stacked separation units would be a potential issue in scaling up the design.
The other limitation of conventional electrophoresis separation is the maximum analyte concentrate (REE ions) in the separation medium. In preliminary results, the total REE concentration never exceeds several tenths of a milligram per liter [1]. Increasing the analyte concentration requires more complexing agent per liquid volume and leads to high electrolyte conductivity and intensifies Joule-heating. Consequently, the energy efficiency of the method will drop, and heavy cooling is required on the plates along the bed. Besides, excessive Joule-heating creates hot spots and promotes bubble formation within the bed which disrupts laminar flow regime. Furthermore, Joule-heating changes physical properties (i.e. fluid viscosity , equilibrium constants and electrophoretic mobilities) and may promote pure-zone broadening, which adversely affects the FFE separation . Therefore, FFE suffers from serious limitations in applications requiring more than several tenths of a grams of analyte per liter.
Following a rigorous literature review and lab work, a combination of multiple resolutions was conceived to address abovementioned challenges to improve REE electrophoretic separation . The approach required a specific separator design, which may not match what is required for FFE. Major modifications could be classified in three groups: (1) Separation medium: Non-aqueous (nAq) medium was selected instead of aqueous. Hence, molar specific conductivity of the concentrated REE solution was significantly improved. Also, REE complexation does not need any buffer or pH control within the chamber. (2) Focusing: A temperature gradient focusing (TGF) is adapted to focus REEs after separation and reduce zone broadening in the chamber. (3) Apparatus: Separation does not occur in a laminar flow regime anymore; thus, separation chamber dimensions are not limited by low Reynolds number, as is the case for FFE.
Method
Non-aqueous Media
Non-aqueous (nAq) electrolyte solution has been studied and applied in chemistry [2]. Solvation and coordination chemistry of three valent metals (e.g. REEs) are significantly different when their salt is dissolved in a nAq solvent [3]. It has been shown that the ionization constants of weak acids and bases are different from that in aqueous solution [4]. Both abovementioned phenomena have a major impact on REE complexation and therefore, electrophoretic mobility variation when applied in electrophoretic separation of REEs [5]. Application of nAq solvent in electrophoresis separation has been subjected to many investigations [6, 7]. However, the advantages of such a system is a controversial topic to date [8–10].
The author observed that the advantage of nAq solvent in this application is twofold. First, REE complexation with carboxylic acids occurs readily, since dissociation constant of weak acids are much less in nAq [9] and their complexes are much more stable. Therefore, complex formation does not require a buffer and precise pH control . Second, the electrophoretic mobility of hydronium is much lower in nAq solution, resulting in a lower electric current under the equivalent chamber dimensions, REE concentration and applied voltage [9]. Consequently, much higher REE concentration can be accommodated in a nAq solution than in an aqueous medium subjected to the same voltage in a similar geometry.
Also, the author observed that in a nAq solution loaded with a single REE salt, specific conductivity is an accurate measure of REE electrophoretic mobility. Such a behavior is not observed in aqueous electrolyte conductivity, which is seriously impacted by the hydronium. Subsequently, the author carefully measured the specific molar conductivity of 4 REE -nitrates in different nAq medium which were complexed with several anionic and neutral chelating agents at 25 °C in the absence of any buffer [11]. The author observed that a carboxylic anion dissolved in an amphiprotic hydroxylic solvent stabilizes REE in concentrations up to 30 g/L, while maintaining sufficient differences in elemental mobilities for an effective electrophoretic separation .
Focusing
The electrophoresis separation is based on the migration and thus, distribution of charged analytes according to their effective electrophoretic mobilities (μi). The effective mobility of analytes depends on their effective charge density, which is calculated based on the complexed ligand-net charge and solvated hydraulic diameter. During electrophoresis migration, although the analytes are separated based on μi, pure zones broaden with time (or space if moving) due to dispersion. Dispersion causes adjacent pure zones to overlap and reduce the effectiveness of the separation .

Variation of net force versus axial position of analyte provides formation of a stagnant equilibrium zone for similar compounds
Adapted from Giddings and Dahlgren [12]
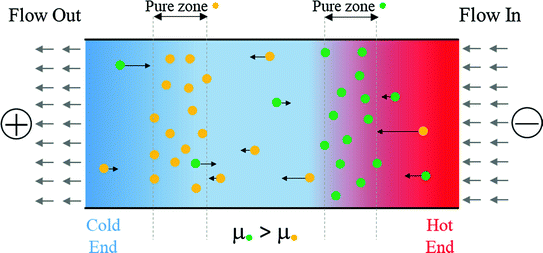
Schematic of a pseudo steady state binary separation of element A•(green) (positive charge) and element B•(yellow) (positive charge) when μ•(green) > μ•(yellow). A creeping counter-flow of solvent provides an opposing convective force field along the separation chamber. Electrophoretic force is attenuated from left to right due to the increasing temperature . Analyte A•(green) migrates and focuses in the hotter zone since it has higher electrophoretic mobility
Apparatus

The scheme of modified Rotofor fractionation system. a Rotofor equipped with 60 ml separation chamber, divided to 20 fractionation cells, filled with REE solution uniformly. b Vacuum harvesting system
Experimental

Modified ROTOFOR (Bio-Rad) assembly for electrophoretic separation of REEs and temperature gradient focusing , INNORD lab, Boucherville, a, b ROTOFOR, c DC Power Supply, d Peristaltic pump as coolant circulator, e Chiller, f Syringe pump which creates counter-flow, g pH/Temp. meter, h Vacuum pump connected to the harvesting system
Results and Discussion
Synthetic equally apportioned lanthanum, europium and ytterbium concentrate were used for the experiments. Separating three elements enabled validating and comparing the results to previous work performed in FFE system [1]. A volume averaged REE concentration over chamber volume is reported since REE concentration is not uniform (Fig. 2) through chamber. Besides, it affects the size of the chamber required for separation of 1 unit mass of REE . In previous work [1] 1 g/L REE solution was injected to the separation channel, carrying a background electrolyte and was diluted to volume average of 3.5 mg/L.

A single pass separation of a synthetic REE -mix in the modified ROTOFOR prototype
Later, the total volume averaged REE concentration was further augmented up to 30 g/L which is over 8000 times higher than previous work (1). The electrophoretic separation of such a concentrated REE solution is several times slower within the same electric field strength. In return, the foot print per kg of REE and energy consumption per kg of REE is significantly reduced.
Conclusion
Electrophoretic separation and temperature gradient focusing of REEs in a rotating cylinder seems to be an adequate separation technique for scaling. The separation prototype demonstrates a significant reduction in footprint as it is several times smaller in comparison with the preceding version. More importantly, separation testing was conducted in a liquid which contained 18 to 800-fold more REE per unit of volume. In addition, power consumption per unit mass of REE has been lowered significantly compared to previous prototype [1]. Moreover, this prototype costs about one tenth of what had been used for FFE [1]. Therefore, increasing the REE concentration and lowering the costs of the separation units were successfully achieved.
This separator works based upon a multi-physics system which includes electro-migration, heat, mass and momentum transfer, which are all strongly interconnect in a time-dependent physics. Therefore, scaling of such a complicated geometry requires a detailed numerical model.
Acknowledgements
This research was partly supported by Industrial Research Assistance Program (IRAP) through the National Research Council Canada (NRC) under project number of 849874.