Top-Down Processing in Art
Reductionist approaches are well defined in science, where they are commonly selected as a strategy for solving a particular complex problem by exploring its simplest representation. Reductionism serves a somewhat different purpose in art, which we shall examine later. This chapter considers the elementary neuronal mechanisms that contribute to top-down processing in art and illustrates how they were delineated by using a reductionist approach.
A striking example of a reductionist approach in biology is the study of heredity. Until the beginning of the Second World War, little was known about the fundamental nature of heredity or even the molecules that distinguish living from nonliving matter. This awkward state of affairs prompted Erwin Schrödinger, a Nobel Prize–winning physicist who had pioneered the study of quantum mechanics, to write a book based on the question, What is life? Specifically, he asked, “How can the events in space and time which take place within the spatial boundary of a living organism be accounted for by physics and chemistry?” (Schrödinger 1944).
Schrödinger
focused on the work of Thomas Hunt Morgan at Columbia University, which had shown that genes are discrete entities on chromosomes. But nothing was known about the physical structure or chemical composition of genes. Particularly perplexing was their dual nature: genes are both stable, so traits can be passed on from generation to generation, and mutable, so they can change and pass those changes on from one generation to the next. Schrödinger speculated that genes contain an “elaborate code-script” that specifies all the future development of the organism. Thus he pinpointed the central task of biology: to solve the mystery of how information contained within the genes of an organism can specify that organism and how that information can be transmitted and altered.
Around the same time that Schrödinger’s book appeared, the journal Genetics carried a revolutionary article by two biologists, Salvador Luria and Max Delbrück. Reductionism had been used successfully in physics, but Luria and Delbrück were the first scientists to apply it to biology. They showed that bacteria—simple single-celled organisms—could be used as model systems to study the complex processes of gene actions and heredity. But this was only the beginning. In 1944, Oswald Avery at Rockefeller University used studies of bacteria to provide the first evidence that the chemical component of genes that is responsible for inheritance is DNA.
In 1952 James Watson, a biologist, and Francis Crick, a physicist who had become fascinated with the biology of the gene after reading Schrödinger’s book, began a collaboration to follow up on Avery’s finding. Watson was convinced that deciphering the structure of DNA would solve the most important riddle of genetics—in fact, the most important question in all of biology: how DNA is copied and how genetic information is transmitted faithfully from generation to generation. Watson and Crick’s subsequent discovery of the double helical structure of DNA indicated how it is copied and transmitted across generations. As Watson put it, the secret of life had been revealed. Several years later, François Jacob and Jacques Monod at the Institute Pasteur in Paris returned to bacteria and worked out a general picture of how genes are regulated—that is, how they are switched on and off. These amazing insights were realized by the application of reductionist strategies to the biology of inheritance.
But what about the biology of the brain? Can a reductionist approach work on something much more complex than the gene? Can it be applied to brain science to address problems of humanistic importance, and can reductionism contribute to our understanding of top-down processes—that is, how our learned experiences and visual associations influence our perception and enjoyment of art? To answer those broad questions, let us focus on the learned associations of top-down processes and examine three more specific questions: How do we learn? How do we remember? And how do learning and remembering relate to top-down processing in our response to works of art?
From a broad humanistic perspective, the study of learning and memory is endlessly fascinating because it addresses one of the most remarkable aspects of human behavior: our ability to generate new ideas from experience. Learning is the mechanism whereby we acquire new knowledge about the world, and memory is the mechanism whereby we retain that knowledge over time. We are who we are as individuals in great part because of what we learn and remember. But learning and memory also play a larger role in human experience.
Despite its discrete nature, learning has broad cultural ramifications. We know what we know about our world and its civilizations because of what we have learned. In the largest sense, learning goes beyond the individual’s acquisition of knowledge to the transmission of culture from generation to generation. It is a critical vehicle for behavioral adaptation and the only vehicle for social progress. Indeed, animals and people have only two major types of mechanisms available for adapting behaviorally to their environment: biological evolution and learning. Of these, learning is by far the more efficient. Changes brought about by biological evolution are slow, often requiring thousands of years in higher organisms, whereas changes produced by learning can be rapid, occurring repeatedly within the life span of an individual.
The potential for learning parallels the complexity of the nervous system. Thus, while the ability to learn and to remember is characteristic of all moderately evolved animals, it reaches its highest form in human beings. In us, learning has led to the establishment of a completely new kind of evolution—cultural evolution—which has largely supplanted biological evolution as a means of transmitting knowledge and adaptations across generations. Our capacity for learning is so remarkably developed that human societies change almost exclusively by cultural evolution. In fact, there is no strong evidence of any biological change in the size or structures of the human brain since
Homo sapiens appeared in the fossil record some 50,000 years ago. All human accomplishments, from antiquity to modern times, are the product of cultural evolution, and therefore of memory.
The biological study of learning raises some familiar philosophical questions: What aspects of the organization of the human mind are innate? How does our mind acquire knowledge of the world?
Serious thinkers in every generation have struggled with these questions. By the end of the seventeenth century, two opposing views had emerged. The British empiricists John Locke, George Berkeley, and David Hume argued that our mind does not possess innate ideas; rather, all knowledge derives from sensory experience and is therefore learned. By contrast, the continental philosophers René Descartes, Gottfried Leibniz, and particularly Immanuel Kant argued that we are born with a priori knowledge; our mind receives and interprets sensory experience in an innately determined framework.
During the early nineteenth century, it gradually became clear that the methods of philosophy—observation, introspection, argument, and speculation—could not, by themselves, either distinguish or reconcile conflicting views about how learning occurs, because the answers would depend upon knowing what goes on in the brain when we learn.
Mind is a set of operations carried out by the brain. For biologists interested in how the brain performs these mental functions, the study of learning has further appeal: unlike thought, language, and consciousness, learning is amenable to reductionist analysis on both a behavioral and a molecular level.
At the beginning of the twentieth century two forms of associative learning were discovered: classical and operant conditioning. Classical conditioning, discovered by Ivan Pavlov, involves an animal or a person learning to associate two stimuli with each other. When a neutral stimulus, such as a tone, is followed repeatedly by a reinforcing stimulus, such as a shock, it causes the animal or the person to learn to withdraw from the neutral stimulus. Operant conditioning, discovered by Edward Thorndike, involves an animal learning to associate a stimulus with a response. If an animal is shown a lever that results in a food reward, the animal will learn to press the lever and will do so progressively more rapidly and efficiently. As a result of these advances, elementary forms of learning and memory were well characterized on a purely behavioral level in both experimental animals and human beings by the first half of the twentieth century. These basic forms of learning represent the most clearly delineated and, for the experimenter, most easily controlled of any mental processes.
At the same time that Pavlov, Thorndike, and B. F. Skinner were exploring the psychology of learning and memory, Alois Riegl and his younger disciples Ernst Kris and Ernst Gombrich were working to establish art history as a scientific discipline by grounding it in psychology. As we have seen, Kris and Gombrich showed that learning and memory are essential to visual perception and thus to our response to art. That response entails not simply seeing a work, but also associating it—through top-down processing—with memories of other works of art we have seen and with other life experiences that the work brings to mind.
Early generations of psychologists thought they could understand learning and memory without understanding the biology of the brain because they believed that these mental processes do not map directly onto the brain. With the emergence of the new science of mind, however, it has become evident that all mental processes are biological processes and that our understanding of any mental process is greatly enhanced by relating its behavioral manifestation to its underlying biological organization. In other words, to answer questions related to the mechanisms underlying learning and memory, we must examine the brain directly. In recent years neuroscience has done just that, and we now have some preliminary answers.
By the 1950s neuroscientists realized that the black box of the brain could be opened. The problem of memory storage, once the exclusive province of psychologists and psychoanalysts, had begun to yield to the methods of modern biology. As a result, studies of memory were increasingly designed to translate some of the central unresolved questions in the psychology of learning and memory into the empirical language of biology. The questions now became: What sort of changes does learning produce in the neural networks of the brain? How is memory stored? Once stored, how is memory maintained? What are the molecular steps whereby a transient, short-term memory is converted to an enduring, long-term memory?
The purpose in attempting this translation was not to replace psychological thinking with the logic of molecular biology, but to contribute to the synthesis of psychology and biology and thereby to forge a new science of mind that would do justice to the interplay between the psychology of memory storage and the molecular biology of cell signaling.
In 1957 the pioneering work of Brenda Milner and her colleagues revealed that certain forms of long-term memory are acquired and encoded by the hippocampus and other regions of the medial temporal lobe, brain structures that are required for conscious awareness. It soon emerged that the brain is capable of forming two major types of memory: explicit (declarative) memory, for facts and events, people, places, and objects; and implicit (nondeclarative) memory, for perceptual and motor skills.
Explicit memory generally requires conscious awareness and relies on the hippocampus, whereas implicit memory does not require conscious awareness and relies mostly on other brain systems: the cerebellum, the striatum, the amygdala, and, in invertebrate animals, simple reflex pathways themselves. Explicit memories are stored throughout the cortex, whereas implicit memories are stored in a variety of brain regions. Thus we call on the hippocampus when recalling our fondest memories or responding to art, but not when riding a bicycle, because riding a bicycle does not require conscious recall.
Milner’s work in the 1970s showed that we have two different types of memory systems, but it was not clear how either of them stores memory. In fact, scientists did not even have a reliable biological context in which to study the mechanism underlying memory storage. At the time, biologists could not distinguish between the two main—and conflicting—theories of memory. One of these, the aggregate field theory, assumed that information is stored in the bioelectric field generated by the average activity of many nerve cells, or neurons. The other, the cellular connectionist theory, posited that memory is stored as an anatomical change in the strength of synaptic connections, the contact points where one neuron communicates with another. The latter theory was derived from the ideas of Santiago Ramón y Cajal, the great Spanish pioneer who was the first person to study the cellular functioning of the brain (Cajal 1894).
It soon became clear that a promising way to distinguish between these disparate theories of memory storage was to take a reductionist approach with a simple animal, which would have so few neurons that nearly all of their interconnections and interactions could be identified. Scientists could then examine the interactions of the individual neurons involved in a simple behavior to see how those interactions were changed by learning and memory storage.
Although reductionist approaches were traditional in many areas of biology, investigators were generally reluctant to consider a reductionist strategy when it came to mental processes such as learning and memory. Yet it was clear that memory storage is so important for survival that its mechanisms are very likely conserved. (A conserved biological process is one that proved so useful in primitive organisms that it has persisted, through evolution, in animals of increasing complexity.) Thus, a molecular analysis of learning, no matter how simple the animal or the task, was likely to reveal universal mechanisms of memory storage.
One animal that seemed almost ideal for a radical reductionist analysis of learning and memory was the large sea snail
Aplysia (
fig. 4.1, left; Kandel 2001). Indeed, the advantages of the snail for a reductionist analysis had been appreciated by Henri Matisse almost a decade earlier. Toward the end of his career, Matisse realized that he could reconstruct the basic visual elements of a snail with only twelve simple blocks of color, which he cut out of paper (
fig. 4.1, right). The purity of color that characterizes Matisse’s last, and perhaps greatest, period has been said by the art historian Olivier Berggruen “to induce in the viewer a feeling of freedom, of liberation from all that is material” (Berggruen 2003). The colored surfaces capture both the essential form and, with some imagination on the beholder’s part, the movement of the snail.
4.1 Left: The marine snail (Aplysia); right: Henri Matisse, The Snail, 1953
Left: Photograph courtesy Thomas Teyke. Right: Tate, London / Art Resource, NY. © 2016 Succession H. Matisse / Artist’s Right Society (ARS), New York
4.2 Comparison of the human brain (left) and Aplysia brain (right). The human brain is numerically complex (100 billion neurons), while the brain of Aplysia is simple (20,000 neurons).
Aplysia’s various behaviors—orienting, feeding, mating, crawling, and defensive withdrawal—are controlled by a very simple nervous system made up of relatively few neurons. While the human brain has 100 billion neurons,
Aplysia has only 20,000 (
fig. 4.2). These neurons are distributed in ten clusters called ganglia, each of which contains about 2,000 cells and controls a family of behaviors. As a result, certain simple behaviors may involve fewer than 100 neurons. This numerical simplicity makes it possible to identify precisely an individual cell’s contribution to a given behavior. Scientists therefore set out to delineate in this simple animal the simplest possible behavior: the gill-withdrawal reflex (Kandel 2001; Squire and Kandel 2000).
Aplysia has an external respiratory organ called the gill, which is covered by a protective sheath known as the mantle shelf. The mantle shelf contains the animal’s residual shell, which ends in a fleshy spout called the siphon. In response to a light touch on its siphon,
Aplysia withdraws its gill. The withdrawal is a defensive reflex, much like pulling your hand back from a hot object (
fig. 4.3). This elemental response is mediated by a very small number of neurons and can be modified by several forms of learning, including classical (Pavlovian) conditioning. This form of associative learning is recruited unconsciously in our brain when we look at a painting and implicitly relate it to other paintings we have encountered or to relevant personal experiences.
Studying the connections among the neurons involved in the gill-withdrawal reflex—that is, the neural circuitry of the reflex (
fig. 4.4)—proved a relatively simple matter. The reflex involves 24 sensory neurons that connect to six motor neurons, both directly and through interneurons (Kandel 2001).
4.3 Aplysia’s gill-withdrawal reflex can be modified by learning. A weak tactile stimulus to the siphon normally causes the gill to withdraw modestly (middle panel). A shock to the tail scares the animal so that the same weak stimulus to the siphon now produces a much more powerful withdrawal of the gill (right panel). The animal remembers the fear induced by the tail shock as a function of the number of training trials: one tail shock leads to a memory that lasts for minutes, whereas five tail shocks produce a memory that persists for days or weeks.
4.4 Neural circuit of the gill-withdrawal reflex. Shocks to the tail strengthen the circuit’s connections through the release of serotonin.
Aplysia’s neural circuit proved surprisingly invariant. Not only does
every animal use the same cells in the reflex circuit, but also those cells are interconnected in precisely the same way in every animal. Each sensory cell and each interneuron connects to a particular set of target cells and to no others. These findings gave us the first insight into a simple example of Kantian
a priori knowledge. They showed that built into the brain, under genetic and developmental control, is the basic architecture of behavior—in this case, the capability for withdrawal from a noxious stimulus. Subsequently, similar invariance was found in other behaviors of
Aplysia (Kandel 2001, 2006).
This insight raised a profound question: How can learning occur in such a precisely wired neural circuit? That is, if the neural circuitry of a behavior does not vary, how can that behavior be modified?
The solution to this apparent paradox is rather simple: learning changes the strength of the connections among neurons (Castellucci et al. 1978; Hawkins et al. 1983). Even though Aplysia’s genetic and developmental program ensures that the connections between cells are correctly specified and invariant, it does not specify the strength of those connections. Thus, much as Locke might have predicted, learning plays upon the basic connections of the neural circuit to form memory. Moreover, persistent alteration in the strength of those connections is the mechanism whereby memory is stored. We see here a reconciliation of nature and nurture, of the Kantian and Lockean points of view, in an elementary and reduced form.
How is memory maintained in the short term and in the long term? To answer these questions, the studies of Aplysia focused on several forms of learning, each of which required the snail to modify its behavior in response to different experimental stimulation. Observing how these changes in behavior came about would reveal the mechanisms of learning.
One form of learning used in these studies was classical conditioning. A light touch to
Aplysia’s siphon causes the animal to withdraw its gill slightly (
fig. 4.3). When a light touch to the siphon is followed immediately by a shock to
Aplysia’s tail, the animal learns that the touch to the siphon
predicts the shock to the tail. A subsequent light touch to the siphon alone will produce a massive withdrawal of the gill. Thus, in classical conditioning
Aplysia learns to associate the light touch to the siphon with the shock that follows it.
What are the molecular underpinnings of this form of memory storage? Studies of the synaptic connections between the sensory and motor neurons that control the gill-withdrawal reflex in
Aplysia revealed that a single stimulus to the tail leads to the activation of modulatory neurons that release serotonin onto the sensory neuron, resulting in an increase in the strength of its synaptic connections with the motor neuron (
fig. 4.4). The release of serotonin leads to an increase in the concentration of a signaling molecule in the sensory cell called cAMP (cyclic adenosine monophosphate). The cAMP molecules signal the sensory neuron to release more of the transmitter glutamate into the synaptic cleft, thus temporarily strengthening the connection between the sensory and motor neurons (Carew et al. 1981, 1983).
In classical conditioning, the light touch to the siphon (the conditioned stimulus) is paired with and applied just before the shock to the tail (the unconditioned stimulus) and produces a greater increase in the gill-withdrawal reflex than either stimulus alone does. How does this come about? The sensory neuron fires an action potential in response to the touch to the siphon, which the animal associates with an imminent shock to its tail. The firing of an action potential increases the amount of cAMP produced by serotonin and thus further strengthens the synaptic connection between the sensory and motor neurons. This more robust connection at the synapse permits a more powerful withdrawal of the gill itself.
The duration of Aplysia’s memory of the paired stimuli correlates with the number of training trials. Following a single paired shock, the animal shows a short-term memory of the event, and the reflex is enhanced for a few minutes. After five or more paired shocks, the animal exhibits a long-term memory that lasts for days to weeks. Thus practice makes perfect, even in snails!
How is short-term memory achieved with one training trial, and how is it converted to long-term memory with five trials?
Figure 4.5 (left) illustrates a single sensory neuron that receives information from the skin of the siphon and connects directly to a motor neuron in the gill. A single shock to the tail activates the modulatory cells that release serotonin, setting off a chain reaction inside the sensory neuron. The release of serotonin leads to a transient strengthening of the connection between the sensory and motor neuron (
fig. 4.5, center). When repeated shocks and repeated release of serotonin are paired with the firing of the sensory neuron in associative learning, a signal is sent to the nucleus of the sensory neuron. This signal activates a gene, CREB-1, which leads to the growth of new connections between the sensory and motor neuron (
fig. 4.5, right) (Bailey and Chen 1983; Kandel 2001). These connections are what enable a memory to persist. So if you remember anything of what you have read here, it will be because your brain is slightly different than it was before you started to read.
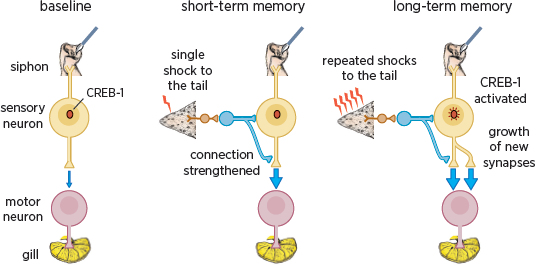
4.5 Different mechanisms underlie short- and long-term memory storage. A single sensory neuron from the siphon skin connects to a motor neuron that innervates the gill. Short-term memory is produced by a single shock to the tail. This activates modulatory neurons (in blue) that cause a functional strengthening of the connections between the sensory and motor neurons. Long-term memory is produced by five repeated shocks to the tail. This activates the modulatory neurons more strongly and leads to the activation of CREB-1 genes and the growth of new synapses.
The mechanisms of associative memory formation (classical conditioning) have proven to be very general. They apply to the vertebrate as well as the invertebrate brain and to explicit, or conscious, memory as well as to implicit, or unconscious, memory (Squire and Kandel 2000; Kandel 2001). These processes of associative memory are called upon in the top-down processing that occurs in our brain when we view a work of art (see
chapter 8).
How important is the growth of new connections among neurons in determining the functional architecture of the human brain? How important is it for you and me? And how does it relate to our response to art?
Figure 4.6 depicts the representation of the surface of the human body in the sensory cortex of the brain, with the most sensitive areas—the hands, eyes, and mouth—being the largest. As we have seen, touch is intimately bound up with vision and is an important aspect of our response to art, particularly abstract art.
Until quite recently, it was thought that this representation, this cortical map, was fixed, but we now know it is not. Michael Merzenich of the University of California, San Francisco, found that, as we saw with the growth of new connections in Aplysia, the cortical maps of an adult monkey are subject to constant modifications on the basis of use. That is, the cortical map is modified by activity in the pathways leading from the various sense organs to the brain (Merzenich et al. 1988). Leslie Ungerleider has shown similar modifications in the human cortical map (Ungerleider et al. 2002).
The brain’s potential for modification appears to vary not only with use but also with age (
fig. 4.7). Thomas Elbert and his colleagues at the University of Konstanz in Germany imaged the brains of stringed instrument players and compared them to the brains of nonmusicians (Elbert et al. 1995). The imaging revealed that the representations of the fingers of the musicians’ left hands, which move independently, often in highly complicated patterns, are significantly larger than those of nonmusicians. Elbert also observed that even though structural changes can occur in the mature brain, such changes are greater in musicians who began their musical training at an early age. Thus the reason the great violinist and child prodigy Jascha Heifetz became Heifetz was not simply because he had the right genes, but also because he began practicing his musical skills very early in life, when his brain was most sensitive to modification by experience.
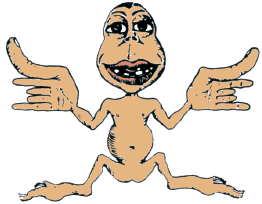
4.6 Somatic-sensory homunculus showing the proportional representation of the surface of the skin in the sensory cortex. Areas of greatest sensitivity are most generously represented in the cortical map.
4.7 Comparison of the size of the cortical representation of the fifth finger of the left hand in string players and nonmusicians (controls). Among string players, those who began playing before age thirteen have a larger representation than those who began later. The horizontal lines represent the mean for each group of dots.
These dramatic results confirmed in humans what animal studies had already revealed in greater detail: namely, the proportion of the cortex devoted to the representation of a specific part of the body depends in part on how much or how little those parts are used.
Since all of us are brought up in somewhat different environments, are exposed to different combinations of stimuli, learn different things, and are likely to exercise our motor and perceptual skills in different ways, the architecture of our brains will be modified in unique ways. We each have a slightly different brain because we have different life experiences. Even identical twins, who have identical genes, will have different experiences and therefore different brains. This distinctive modification of brain architecture, along with our distinctive genetic makeup, constitutes the biological basis for the expression of individuality. It also accounts for differences in how we respond to art. As we have seen, our response depends not only on innate, bottom-up perceptual processes in art but also on top-down associations and learning, which are mediated by changes in synaptic strength.
The reductionist approach to brain science has revealed that learning leads to changes in the strength of connections among neurons. That insight has given us our first clues to how aspects of top-down processing occur. In addition, we now know a bit about where it occurs: learned visual associations are consolidated in the inferior temporal cortex, a region of the brain that interacts with the hippocampus, which is concerned with the conscious recall of memories. We also understand the basis of our strong emotional response to color and to faces in art: the inferior temporal cortex, which contains specialized regions for processing information about color and about faces, exchanges information with both the hippocampus and the amygdala, which orchestrates emotion.
Emotions—
sexuality, aggression, pleasure, fear, and pain—are instinctive processes. They color our lives and help us confront the fundamental challenges of avoiding pain and seeking pleasure. We shall examine later the interplay of sexuality and aggression in Willem de Kooning’s
Woman I (
fig. 7.5) and Gustav Klimt’s
Judith (
fig. 7.7). The emotions we bring to bear on a painting are essentially the same as those we bring to bear on everything else in our everyday life. Thus art challenges us to develop new insights into brain science by posing numerous questions about perception and emotion that we are only beginning to appreciate and address. In particular, we are now in a position to begin to explore how different emotional states influence our perception of figurative, as opposed to abstract, art.
While we are just beginning to understand how our brain mediates our perception and enjoyment of art, we do know that our response to abstract art differs significantly from our response to figurative art. And we know why abstract art can be so successful. By reducing images to form, line, color, or light, abstract art relies more heavily on top-down processing—and therefore on our emotions, our imagination, and our creativity. Brain science offers us the potential to develop additional insights into the role of top-down perception in art and into the creativity of the beholder.
Having considered reductionist strategies in biology that pertain to top-down processing, let us now turn to art. Here, reductionist strategies have also been used, consciously or unconsciously, in a variety of different ways.