Chapter 9 No Silver Bullet
The pundits were united in their scorn—surely, this time, Elon Musk, the provocative CEO of Tesla Motors, had gone too far. On June 21, 2016, Tesla had announced plans to acquire SolarCity, the dominant player in the residential U.S. solar market but a company with no apparent connection to Tesla’s electric car market. Tesla’s shares plunged by as much as 10 percent as analysts panned the deal. One prominent investor even called the deal “shameful.”1
The dominant narrative was that SolarCity, whose business model required fronting huge sums of capital to finance rooftop solar systems that homeowners would pay back over time, had been hemorrhaging cash and needed a bailout. Already, SpaceX—which Musk also helms—had lent hundreds of millions of dollars to SolarCity, which was run by Musk’s cousins, Lyndon and Peter Rive. Now, analysts fumed, SolarCity’s sale to Tesla would constitute a bailout within the family. On top of this, Tesla was facing production delays for its new Model 3 mass-market car, and an autonomously piloted Tesla Model S had just crashed, killing its driver. How could this be the right time to merge with an embattled firm from another industry?
Musk actually agreed with his critics that the timing wasn’t ideal. But wryly, he quipped that the merger “may even be a little late.”2 As obviously disgraceful as the proposed sale was to the pundits, combining the two companies had been just as obviously a no-brainer for Musk for over a decade. In 2006, Musk published “The Secret Tesla Motors Master Plan” on his blog, promising to build an expensive electric vehicle (EV) for early adopters, use the proceeds to fund development of a cheaper EV for the masses, and in parallel produce cheap, zero-emission solar power.3
Now, the Tesla/SolarCity merger was a logical culmination of a single, overarching goal. What united both companies was Musk’s drive to transform the way that the world produces and consumes energy. Solar power was a crucial component of that vision, but it was incomplete on its own. Only paired with energy storage—which Tesla Motors conveniently sold in the form of EVs and home batteries—could solar power actually meet most of a household’s energy needs.
Confident in this logic, Musk shrugged off the opposition to his merger plans. He’d heard this type of criticism many times before. For a man who thinks decades and centuries ahead, Musk was never likely to be fazed by day-trader hysterics. In addition to plotting a transformation of the world’s energy systems, he has set his sights on building reusable launch vehicles and spacecraft to colonize Mars. What sets him apart from other dreamers is an intimate familiarity with the details of his various ventures—from personally leading the investigation into faulty tanks of supercooled propellant that caused a SpaceX rocket to explode in 2016 to insisting on the specifications of retractable door handles on Tesla EVs to minimize their aerodynamic drag.4
This approach has led to astounding results. Among many other milestones, SpaceX won the race to land and relaunch a rocket, and Tesla cars have become so popular that over 300,000 eager buyers put down deposits within a week of the Tesla Model 3 announcement in 2016.
Still, Musk would need his investors to see the future in the way that he did—and it would help to restore the public hype over his companies and vision. So in October 2016, a couple of weeks before shareholders were due to vote on the proposed merger, Musk unveiled a stunning new product, hidden in plain sight.
At an event in Universal Studios, on the set of the television show Desperate Housewives, Musk interrupted his own speech to ask the audience if they noticed anything funny about the houses on the set. To the crowd’s disbelief, it turned out that the houses—elegant but otherwise ordinary to the eye—actually had roof shingles and tiles that concealed silicon solar photovoltaic (PV) cells. What’s more, these solar roofs would cost less than ordinary roofs (although that’s a bit of hyperbole, given that Tesla’s solar roofs aim to replace such materials as terra cotta and French slate, which can be more than twenty times as expensive as ordinary asphalt shingles). To underscore his thesis that SolarCity’s products belonged under (or on) the same roof as those of Tesla Motors, Musk opened a garage door to reveal gleaming Tesla EVs and the company’s Powerwall home battery. The tableau was a vivid depiction of Musk’s vision of the future, in which solar panels and batteries work hand in hand to eliminate a household’s carbon footprint—all in considerable style.
Predictably, aversion turned to adulation. Shareholders overwhelmingly approved Musk’s proposed merger, and they were repaid handsomely. The combined firm promptly soared in value, and by April 2017, it reached a market cap of over $50 billion—more valuable than any U.S. car company.5 On its website, Tesla Motors quietly changed its name to just “Tesla,” signaling to the world that the ultimate energy company had arrived—it wasn’t just a car company anymore.
Musk is betting the house—solar roof tiles and all—on his hypothesis that energy storage is crucial to enabling a solar-powered future. In particular, he is betting on a particular energy storage technology: lithium-ion batteries. Although these batteries were almost exclusively used in laptops and other consumer electronics a decade ago, Tesla is deploying them en masse not only to power its vehicles, but also as stand-alone battery packs, sized for individual households, or even as large-scale backup resources for the power grid.
Musk believes that he can re-create the dramatic cost declines achieved in solar by similarly scaling up the production of lithium-ion batteries. So out in the Nevada desert, Tesla has built its first Gigafactory, which aims to produce more lithium-ion batteries in 2018 than the entire world had produced just five years earlier. Word of the facility’s size has sparked a lithium-ion arms race, as rivals from China (BYD) and South Korea (LG Chem and Samsung) have ratcheted up battery production in anticipation of the Gigafactory. All this activity assured that Musk’s prediction of falling battery costs would be a self-fulfilling prophecy. Indeed, in 2016, the cost of a battery pack plunged, going from more than $400 per kilowatt-hour (kWh) of storage capacity to less than $200/kWh (figure 9.1).6
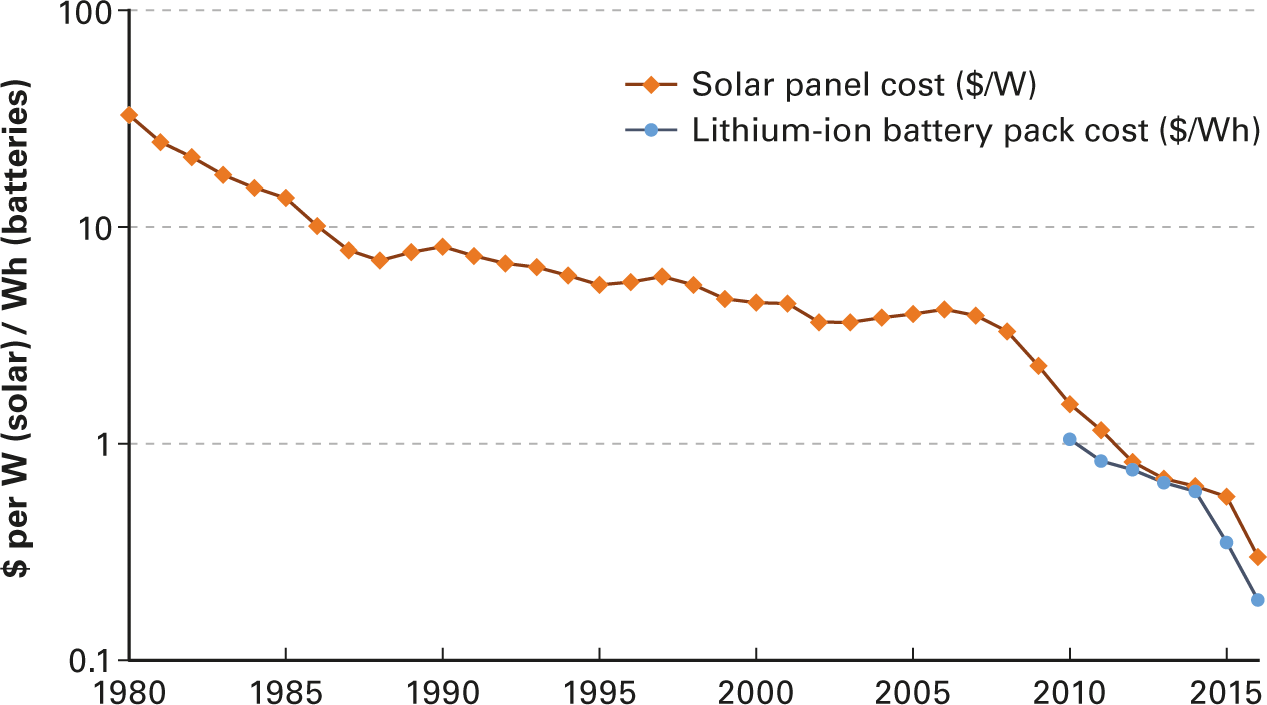
Comparison of lithium-ion battery and solar PV panel costs. This chart plots the falling cost of a solar PV panel, expressed in dollars per watt of power-generating capacity; and that of a lithium-ion battery pack, expressed in dollars per watt-hour of energy-storage capacity. (The cost of solar panels is just one component of the cost of a fully installed solar PV system; similarly, the cost of battery packs is just one component of a fully installed battery installation). The y-axis is logarithmic.
Source: Bloomberg New Energy Finance, industry reports.
The logic of using battery storage to enable a high penetration of solar power is seductive. After all, combining the two technologies would yield a perfectly flexible power source, freed from the intermittency of sunlight. And if batteries were to get cheap enough, most, if not all, of solar’s flaws would appear to vanish. Paired with batteries, solar PV would no longer be afflicted by economic value deflation because, instead of being worth nothing, any excess power output from solar panels at sunny times could be stored and sold to the grid during periods of peak demand. Power grids would no longer strain to accommodate intermittent solar power and match supply and demand—the batteries would handle that. And if Musk succeeded in popularizing mass-market EVs, a grid powered by solar panels and buffered by batteries could loosen oil’s viselike grip on transportation by charging up EVs.
Unfortunately, although pairing solar with grid-tied battery storage may be cognitively satisfying, it is no silver bullet. The combined cost of solar PV and lithium-ion batteries still exceeds that of a conventional power plant fueled by natural gas. Were that combined cost to plummet even faster than existing forecasts predict, lithium-ion batteries would still be ill-suited for storing large amounts of solar power for long durations—such as days, weeks, or even months. The need for such long-duration storage arises because of seasonal differences in how much solar energy strikes Earth, for example between winter and summer.
That lithium-ion batteries are ill-suited to long-term, mass storage shouldn’t be surprising, given that they are great for powering your smartphone. Different applications demand different performance characteristics from a storage technology, such as how long it will live, how much energy it can store, and how fast that energy can be charged or discharged. A whole portfolio of storage technologies exists, including many different flavors of battery, pumped hydro storage, compressed air storage, and more. Each of these has different comparative advantages, whether powering an EV, balancing the power grid’s instantaneous imbalances between supply and demand, or storing energy for the long haul. And brand new technologies at the lab bench hold promise to perform each of these functions better than existing products.
In addition, there are still more ways to store energy so as to add flexibility to otherwise inflexible, intermittent solar output. For example, if other power generators could adjust their output rapidly to compensate for fluctuating solar output, this ability would be equivalent to adding storage, at a fraction of the cost.
Indeed, a rich variety of academic studies has demonstrated that the cheapest way to zero out carbon emissions from the global electricity sector is to surround renewable energy with a mix of other resources, including storage, but also nuclear reactors and fossil-fueled power plants whose emissions are captured and stored. Some academics and environmentalists, however, prefer to rule out such a diverse power mix, recommending instead that countries rely solely on renewable energy and storage. That constraint would be foolhardy and disastrous. Jesse Jenkins at MIT points out that powering the United States with just renewable energy and battery storage would require “37.8 billion Tesla Powerwall 2.0 home energy storage systems.”7
Rather, for solar to take center stage and achieve its midcentury target of powering a third of global electricity demand, it will need a truly diverse supporting cast. Unfortunately, power markets around the world are not set up to accommodate a diverse power mix. Because of how these markets work, solar and wind generators are pushing down wholesale power prices and driving more reliable generators like nuclear plants out of business. Reversing this trend will require markets to carefully value the different dimensions of flexibility that the grid will need so as to integrate large servings of solar. Reformed markets could induce investors to build reliable, flexible power plants to complement solar. They could also encourage developers of solar installations to upgrade the electronics that connect solar to the grid so solar can be part of the solution rather than just the problem.
Given the desirability of a diverse power mix, when Musk boasts that with Tesla battery packs backing up homes and power grids, “you can basically make all electricity generation in the world renewable and primarily solar,” he is not describing an affordable, realistic future. But when he convinced his shareholders that merging an electric car company with a solar company made perfect sense, he was on to something more promising. That’s because connecting the transportation and electric power sectors is an important step en route to reaching the long-term target of using the sun to power a majority of humanity’s energy needs by century’s end.
In the future, EVs could act as mobile batteries for the grid, intelligently charging and discharging to compensate for intermittent solar power on the grid without drivers even noticing. The next step beyond linking the transportation sector is to add the heat sector. Homes, commercial buildings, and industrial facilities all demand heat, but they can do so flexibly, thus providing effective storage of fluctuating solar energy output.
The linkages don’t have to end there. Solar can meet pressing needs even outside the energy sector. For example, solar could power desalination facilities to provide fresh water, which will be increasingly scarce as climate change accelerates. Solar also could power a range of agricultural needs and make it possible to preserve food that otherwise would be wasted. In addition, these newly linked sectors could, in effect, provide storage of solar power by putting surplus output to valuable use.
The lesson here is that solar needs storage to thrive—but that storage can come in a myriad of different forms. Elon Musk has presented some of them to the world with his customary panache. Now it’s time for other innovators to step up with equally bold ideas to complement Musk’s vision.
A Class of Its Own
For all the hype over lithium-ion batteries, it might come as a surprise to many that virtually all the energy storage that exists today around the world does not actually take the form of electrochemical batteries. Rather, pumped hydro storage facilities—which pump water from a lower reservoir to an upper reservoir to store energy and release it by letting the water flow back downhill to run a turbine—account for 95 percent of global energy storage capacity.8 It is also not clear that lithium-ion batteries will, or should, ever dominate the energy storage landscape in the way that pumped hydro has to date. Rather, a whole class of various storage technologies exists that offers a range of solutions to the world’s diverse storage needs.
Asking which storage technology is best, or even cheapest, is a meaningless question. There are too many different performance attributes of storage, each of which matters for different applications. Figure 9.2 lays out the landscape of different storage technology categories, plotted according to two of the most important performance characteristics: power capacity and discharge time. Technologies with high power capacity can provide a surge of instantaneous power. And technologies with long discharge times store a lot of energy and can thus provide a constant supply of power for hours, days, or longer. Pumped hydro storage is in the upper-right portion of the graph in figure 9.2, indicating that it can deliver a lot of instantaneous power and sustain that output for long periods of time, which is why it provides almost all the energy storage for power grids around the world, backing up massive power plants and compensating for swings in demand from megacities.

Overview of various electricity storage technologies and attributes. This illustrative chart assesses energy technologies by how much power they can instantaneously supply or store (x-axis) and for how long they can do so, a measure of energy-storage capacity (y-axis).
Source: Energy Information Administration (EIA).
Not every application of energy storage requires high power capacity and discharge time, though. In fact, storage can help stabilize the power grid at a variety of power and time scales. For example, to guarantee that alternating current (AC) electricity is of high quality—that is, has desired and stable voltage and frequency—a grid operator might turn to storage resources that react within milliseconds to charge or discharge small amounts of power for just a few seconds. That need falls in the lower-left corner of figure 9.2. Other needs include filling the gap between supply and demand while a slower generator, perhaps fueled by natural gas, ramps up. This kind of use might require megawatts of power capacity over a few minutes. Still another need, which falls in the upper-right corner of figure 9.2, is to shift generated power from one period of the day, when demand is low, to another period, when it is high—requiring a high power capacity and hours of discharge time.9
Just as the grid’s needs are all over the map of figure 9.2, so too are the sweet spots of various different storage technologies. Some of them are great at producing bursts of high power. These include good old flywheels, which are just spinning masses that store energy in the form of mechanical rotation (one is on the front of your bike in spin class). Newer technologies include supercapacitors, which look similar to batteries and store electrical charge along a highly corrugated surface, and superconducting magnetic energy storage (SMES), which stores energy in the magnetic field created by the current in an ultracold, superconducting coil of wire.
Electrochemical batteries—which store energy in the form of chemical bonds—take up a big swath of figure 9.2. Their output ranges from small to medium-sized power flows that can last anywhere from seconds to hours. But that big, blue region obscures the diversity of battery types. In addition to familiar lead-acid (car) batteries and increasingly popular lithium-ion batteries, others like sodium-sulfur and flow batteries have their own advantages, like the ability to store substantial amounts of energy to discharge over several hours.
Lithium-ion batteries have become the fastest-growing, most-hyped storage technology for several reasons. Compared with other commercially available technologies, lithium-ion has a reasonably high energy density—that is, it packs a lot of energy per unit of mass—which is why it has come to dominate consumer electronics and then EVs. Lithium-ion batteries are also quite reliable in charging and discharging. On the other hand, they can be flammable—witness Samsung phones catching fire on flights in 2016—and their performance can degrade over several hundred discharge cycles.
Researchers are also pushing the performance limits of lithium-ion batteries. The label “lithium-ion” encompasses a whole class of battery chemistries that all charge and discharge by shuttling lithium ions from one side of the battery to the other but can vary in their exact constituents. Elon Musk and Tesla use one of the oldest designs, fashioning one end of the battery (the “cathode”) out of nickel, cobalt, and aluminum (NCA). A more recent version, the nickel-manganese-cobalt (NMC) battery, found its way into the Chevy Bolt, the 238-mile range, mass-market EV that beat Tesla’s Model 3 to market in 2017.
Whether lithium-ion batteries will ultimately prove to be the best solution to power EVs is still up in the air (although studies suggesting that they are close to their energy density ceiling are discouraging because longer-range, affordable EVs will require more energy-dense batteries).10 Certainly, though, they cannot meet the full range of the power grid’s needs. For one thing, they suffer from their own form of value deflation. These batteries are best suited to providing short bursts of stored energy to meet brief spikes in energy demand. But the more batteries that are connected to the grid, the fewer spiky demand peaks there will be to shave, degrading the value of the marginal battery addition.11
As solar penetration increases, the grid will need much more than just smoothing brief spikes in demand. But lithium-ion batteries are unsuited for many of those needs because installations of them tend to be able to provide only up to four hours of storage—less than the duration needed to buffer the variation in solar output between day and night or on longer timescales.
The reason for the time limit may not be obvious to a casual observer. After all, lithium-ion batteries come in modular units that look just like the cylindrical batteries in your television’s remote. It may seem logical, therefore, to assume that stacking enough of the batteries together could shift the output of a large, utility-scale solar farm’s output by, say, six hours to match an evening spike in customer demand. Such a move might, in fact, be physically feasible but is economically unviable. Lithium-ion batteries have a fixed ratio of energy and power density—the bigger the stack of batteries, the more power that they can instantaneously discharge and the longer they can do it. A battery installation sized to discharge for over four hours will end up underutilizing its power capacity, and underutilization prevents the project developer from paying off the high up-front capital cost of the batteries.
A completely different kind of battery—the flow battery—might be a better solution for storing solar power for several hours, or even days. Flow batteries stow energy in the form of liquids that charge and discharge by exchanging ions across a membrane. In a big advantage over lithium-ion batteries, the amount of energy stored in a flow battery can be increased almost without limit just by adding more tanks of liquid, without changing the power capacity. Because its power capacity is decoupled from the discharge time, a flow battery can be sized to cost-effectively store intermittent renewable energy for long durations to help the grid balance supply and demand. Moreover, flow batteries have much longer lifetimes than lithium-ion batteries.
At the moment, flow batteries are a less mature—and hence more expensive—technology than lithium-ion batteries, and they rely on rare elements (like vanadium).12 Reliance on scarce materials should be a red flag for a storage technology with any hope of backing up massive amounts of solar power around the world. (Lithium-ion batteries likewise face issues with scarcity of materials because of their reliance on cobalt, which is one of the world’s most expensive metals and is found primarily in war-torn Congo.)13
To sidestep the high cost and messy risks of resource scarcity, researchers are trying to make inexpensive flow batteries out of Earth-abundant materials, although they are a long way from commercializing a product.14 Others have opted against both the flow battery and lithium-ion battery designs but ensured that their alternatives use Earth-abundant materials. For example, the MIT spin-out Ambri manufactures a liquid-metal battery that could cost-effectively provide several hours of energy storage and has a much longer lifetime than lithium-ion batteries.15
These developments are steps in the right direction toward cost-effective storage that can buffer the daily fluctuations of solar energy. But a whole portfolio of existing and new technologies will likely be required to achieve this at scale. Lithium-ion batteries, complemented by such high-power-capacity technologies as supercapacitors, might be able to smooth solar volatility over seconds or minutes (such as when clouds pass overhead, briefly blocking the sun). They might also shift solar’s output within a couple of hours to better line up with demand. But innovative products made of Earth-abundant materials will probably be needed to shift solar’s output over longer time scales.
Unfortunately, the inexorable rise in manufacturing scale of lithium-ion batteries threatens to lock out new, contender technologies, just as silicon solar panels have locked out subsequent generations of solar materials.16 In a repeat of the solar manufacturing glut a decade ago, the production arms race among lithium-ion battery producers is on track to reduce costs through enormous scale and oversupply, while creating a moat around the industry that emerging technologies cannot cross. And just as it is dangerous to bet on a single solar horse, so too is it suboptimal to bet only on lithium-ion batteries and risk shutting out other technologies that could enable greater solar penetration.
More broadly, it is dangerous to bet even on the entire class of storage technologies to do all the heavy lifting of integrating solar power. Certainly storage has an important role to play—but not the only role. This limitation is clearest when examining the seasonal variation in solar output, which any form of storage would struggle to cost-effectively smooth out. In several parts of the United States, for example, the average solar radiation in the winter is less than half of that in the summer. Because seasonal customer demand may offset only some—or none—of that variation, massive amounts of solar power would need to be stored in the summer months and discharged in the winter months in a scenario with high solar penetration and no other options to balance supply and demand. The cost of seasonal storage would be prohibitive—possibly several times more than the entire existing power system.17
Today, there is really only one commercially available technology capable of providing seasonal storage: pumped hydro storage. But in many parts of the world, including the United States, it is exceedingly difficult to site new, cost-effective hydro facilities in an environmentally friendly way, so a massive buildout is unlikely. Moreover, seasonal storage is an extremely uneconomical way to run a storage resource. Storing energy in the summer and discharging it in the winter cycles the storage resource only once a year, making it virtually impossible to earn enough revenue to defray its capital costs.
Large-scale compressed air storage—in which electricity is used to compress ambient air for storage in an underground cavern and is recovered by expanding the air to run a turbine—is another example of a technology that might provide seasonal energy storage. Yet its viability is hampered by the limited availability of appropriate geological storage caverns. Some researchers and firms have proposed surprisingly low-tech but intriguing ideas, such as having a train lug concrete slabs uphill to store potential energy. It remains to be seen, however, whether those ideas will successfully leave the station.18
All of this means that grid-scale energy storage technologies cannot be the only solution for coping with the intermittency of solar energy. Successful energy systems will require both storage and other ways to adjust electricity flows flexibly. Chapter 8 proposed extending the geographical reach of the grid and creating a smart, decentralized grid to enable responsive demand—both of which are important tools to ease the integration of solar power. But there are many more puzzle pieces that could add further flexibility. Truly transforming the world’s energy systems this century will require taking advantage of all of them.
Getting to Deep Decarbonization
I recently learned that such a logical conclusion can run afoul of politics and preferences. Proceedings of the National Academy of Sciences of the United States of America (PNAS) is one of the world’s most highly respected scientific journals. Each year, it honors six exemplary articles from disparate fields. In 2015, one of the awards went to an article whose lead author was a Stanford professor of atmospheric science, Dr. Mark Jacobson. Entitled, “Low-Cost Solution to the Grid Reliability Problem with 100% Penetration of Intermittent Wind, Water, and Solar for All Purposes,” the paper claimed to lay out a way to power the entire United States only with existing technologies.19 The best part was that this system would be cheaper than using fossil fuels—and just as reliable.
This proposal caused a stir in the popular media, thanks to the enthusiastic stamp of approval from a prestigious journal. It all sounded like a dream scenario—power from all-natural sunshine, wind, and water, without any need for dangerous nuclear reactors or complicated capturing and storage of carbon emissions from fossil-fueled plants.
Unfortunately, this dream scenario was just that: a fantastical dream. As it turned out, the paper was filled with implausible assumptions, and its headline conclusion was almost certainly erroneous. Instead of a “low-cost solution,” the paper’s proposal would be astronomically expensive and complicated. It would require adding hydroelectric generation capacity equivalent to 600 Hoover Dams. It would take trillions of dollars of investment in new electricity transmission lines and infrastructure to produce, transport, and store hydrogen. It would demand an expansion of solar and wind power capacity well in excess of any buildout of power plants that the world has ever seen.20 And it would require underground thermal energy storage—a technology to store heat in groundwater or bedrock underground that is unproven at any meaningful scale—beneath “nearly every home, business, office building, hospital, school, and factory in the United States.”21
That last line is a quote from a rebuttal in the same journal, which I wrote with twenty of the scholars I respect most in the fields of energy science, economics, and policy. We thought that the Jacobson paper created a dangerous illusion that today’s renewable energy and energy storage technologies could cost-effectively decarbonize the world’s energy systems. On top of this, the paper excluded viable options like nuclear power—effectively tying one arm behind the back.
The day after we published our rejoinder, the front page of the New York Times business section reported “Fisticuffs over the Route to a Clean-Energy Future.” Other media outlets declared a “Battle Royale,” a “Bitter Scientific Debate,” and “One of the Most Vitriolic Fights in Science Publishing.”22 And my coauthors and I were accused of being fossil-fuel shills by no less an expert than Mark Ruffalo (the actor who plays the Incredible Hulk). The episode underscored that the topic of how the world slashes its carbon emissions is politically fraught, even in the supposedly apolitical realm of academia.
Politics aside, getting to near-zero emissions—or as insiders call it, “deep decarbonization”—in the power sector is a crucial prerequisite to limiting climate change, and it will be an uphill battle even with all options on the table. A survey of eighteen different models of the global economy concluded that to halt runaway carbon emissions in the atmosphere, emissions from electricity would need to fall by between 80 and 100 percent by 2050, and emissions from all other sources would need to vanish by 2100.23,24 The urgency behind cleaning up the power sector is that many other uses of energy—including aviation, shipping, heavy-duty trucking, and fertilizer production—are tougher or impossible to decarbonize.25 So it is crucial to apply existing and emerging technologies to slash emissions in the power sector, while electrifying as many other uses of energy—among them, passenger vehicle transportation—as possible. Down the road, it will also be important to find clean fuels to replace oil to zero out emissions from more stubbornly dirty sectors. It may even be necessary to figure out how to suck carbon out of the air. Decarbonizing power is the least difficult of these monumental tasks, so it makes sense to start there.
Complicating the path to deep decarbonization of the power sector is the pesky fact that eliminating the first 10 percent tranche of emissions is much easier than eliminating the last one. For example, thanks to the favorable economics of switching from coal to natural gas–fired power (whose emissions are only half those of coal), U.S. emissions fell by several percentage points from their peak in 2007.26 But reducing emissions from natural gas plants, for example by capturing and storing them, is hugely expensive at present. Similarly, the first solar panel on a grid can displace some fossil-generated power without disrupting the balance between supply and demand. But a high penetration of solar power leads to skyrocketing costs to accommodate its intermittency.
Hence, a power system can easily get stuck in a cul-de-sac off the highway to deep decarbonization. For example, a review of decarbonization studies concluded that steadily increasing renewable energy, switching from coal to natural gas, and just maintaining existing nuclear and hydropower capacity could reduce power-sector emissions by over 50 percent.27 But reducing emissions by 80–100 percent would require a surge in new nuclear reactors, carbon capture and storage setups for almost all fossil plants, and an explosion of new solar and wind capacity.28 It is crucial, then, to start planning and investing today in a power system with all the right elements to reach deep decarbonization decades later.
In such a system, three broad classes of power supply are needed. The first is renewable energy, mostly from wind and solar power. But because these sources are intermittent, much more reliable power is needed, from what Jesse Jenkins at MIT calls “flexible-base” resources. These include nuclear reactors and a type of natural gas power plant known as a “combined-cycle gas turbine,” which is the most efficient type of gas plant. (For natural gas plants to contribute to a decarbonized power system, they would need to be equipped with carbon capture and storage). The flexible-base plants would consistently pump out power but would be capable of cranking their output up or down to compensate for fluctuating renewable energy. Still, because these plants might not be able to immediately adjust their output in response to capricious solar and wind generators, a third class of fast-acting resources would fill the gaps and provide surges of power during rare spikes in customer demand. This third class might include another type of natural gas plant—an “open-cycle gas turbine”—that is less efficient than a combined-cycle gas turbine but can change its power output more quickly. Other fast-acting resources include batteries and demand response systems (possibly controlled by a grid hive mind as discussed in chapter 8).
No two of these classes can cost-effectively meet customer demand without the third. Figure 9.3 illustrates the challenge of trying to cut out flexible-base resources and power the United States using only renewable energy and energy storage resources. Doing so would require enough batteries or other storage capacity to hold between eight and sixteen weeks worth of all U.S. electricity consumption—an absolutely massive quantity. For some perspective, the United States currently has storage equivalent to about forty-three minutes of its power consumption, and nearly all that storage is in the form of pumped hydropower.

Estimates of battery storage capacity needed to power the United States with 100 percent renewable energy. The top row represents the amount of stored electricity that would be needed to supply one week of national electricity demand if no other supply sources were available. The remaining four rows display the estimates from each of four academic studies of the amount of energy-storage capacity that would be needed to ensure that U.S. electricity demand were reliably met in the event that all electricity supply came from renewable sources.
Source: Jenkins and Thernstrom (2017).
Adding flexible-base resources is the much saner route, and this lesson applies around the world. For example, a study of China’s power system concluded that the cheapest route to reducing emissions by 80 percent by 2050 entails an even mix of renewable energy and flexible base, comprising nuclear reactors and coal plants equipped with carbon capture and storage.29 Although some studies—including careful ones by the National Renewable Energy Laboratory (NREL) and the Climate Policy Initiative—suggest that the share of renewable electricity could get all the way to 80 percent, they also maintain that flexible base would still play an essential role.30,31 Other studies that purport to demonstrate the feasibility of relying on 100 percent renewable power sources would find far lower power system costs if they removed their artificial constraints on which zero-carbon power sources are allowed.
My collaborators at MIT and GTM Research and I have done just that: We simulated the Texas power grid without arbitrarily excluding any source of power. Predictably, we found that the most economical penetration of solar PV rose as it got cheaper (figure 9.4). But no matter how cheap solar got, flexible-base resources—not solar—provided a majority of the system’s electricity. Then, we really stacked the deck in solar’s favor, imagining a dream scenario in which batteries were four times cheaper than they were in 2016 (we assumed a cost of $150/kWh for a fully installed battery system, even though such a system is projected to only fall in cost to $280/kWh by 2040).32 Yet, even with the option to store energy in extremely low-cost batteries, the economical penetration of solar inched up by only around 5 percent—flexible base was still indispensable. Thus, batteries have a limited—albeit important—role to play in enabling greater solar penetration.
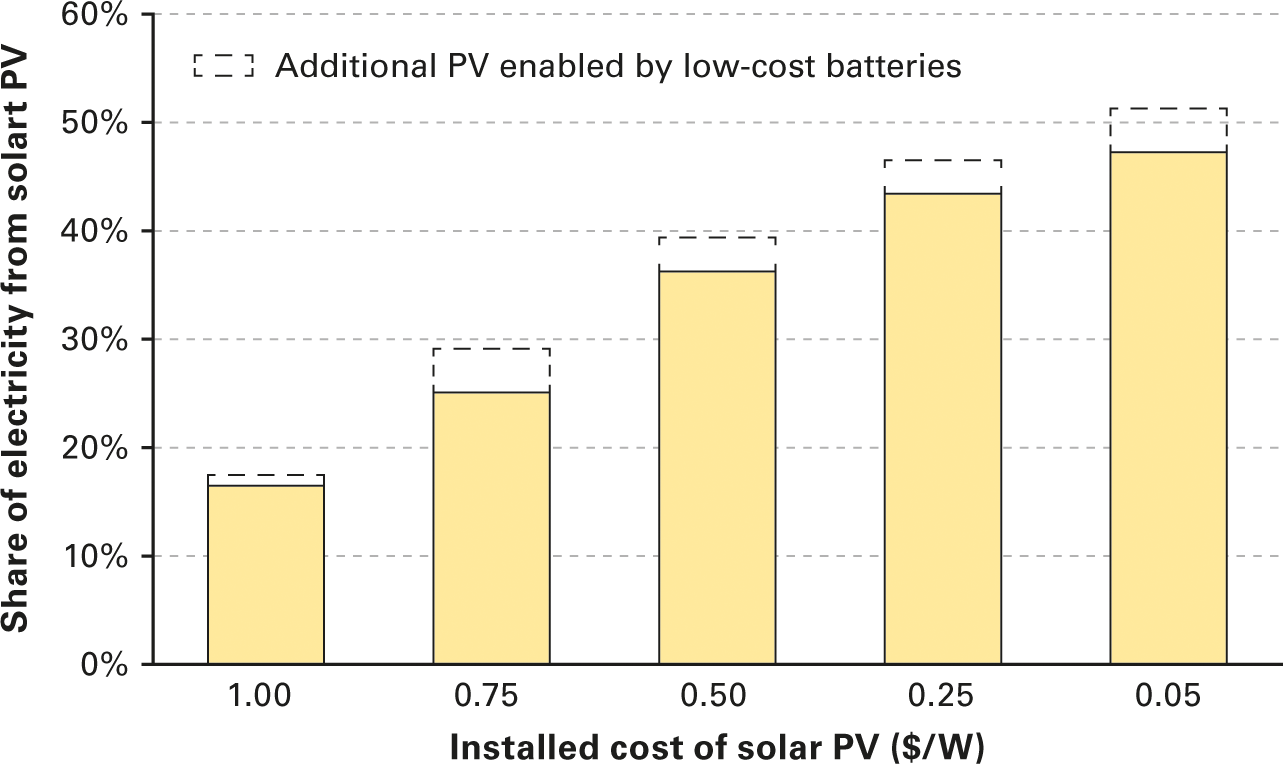
Grid penetration of solar PV as a function of PV and storage costs. For each of the assumptions of the installed cost of solar PV along the x-axis, the filled bars represent the proportion of total electric energy, measured in kilowatt-hours (kWh), that solar PV would generate. The dotted extensions above the bars illustrate the additional amount of electricity that would be economical to generate with solar PV if a fully installed battery system cost only $150/kWh. These results were obtained by simulating a region with similar weather, grid transmission capacity, and electricity demand to Texas and then determining the cheapest set of power-generating resources to reliably meet demand.
Source: Brown, Jenkins, Sepulveda, and Sivaram (2017).
Still, it will take innovation to deliver zero-carbon, flexible-base plants, such as advanced nuclear reactors. Importantly, this is not because today’s nuclear plants are technically incapable of operating flexibly (a common misconception). Actually, nuclear plants can ramp their output up and down to help the grid balance supply and demand, as they have done in France for decades.33 Rather, the trouble with today’s nuclear plants, known as light-water reactors, is that they are expensive and—in extremely rare circumstances—vulnerable to meltdowns or other accidents.
The 2017 bankruptcy of Westinghouse, a nuclear pioneer that faced mounting costs and delays to construct its plants, has dimmed the prospects for new nuclear reactors in the United States. But newer designs (actually based on ideas shelved decades ago), known as “Generation IV” nuclear reactors, could be both cheaper and safer. Unfortunately for the United States, China and Russia are leading the race to commercialize advanced nuclear reactors, while America lags mostly because of limited government research and development (R&D) investment and outdated regulations that discourage new plant designs.34
Similarly, carbon capture and storage technologies need to become vastly cheaper to enable fossil-fueled plants to provide zero-carbon, flexible-base power. The historical trend of carbon capture and storage demonstration projects ballooning in cost is not encouraging. Some cite this pattern as evidence for the superiority of an all-renewable solution, and they argue that betting on carbon capture and storage might open the door for the continued burning of fossil fuels. Then, if cost-effective carbon capture and storage technology does not materialize, the world will be locked in to fossil fuel infrastructure.
Two developments in Texas suggest that carbon capture and storage does not have to be impossibly expensive. First, through the Petro Nova project, the power producer NRG has retrofitted one of the largest coal plants in the United States—the WA Parish Generating Station outside Houston—with equipment to capture its carbon dioxide emissions. The project was completed on time and on budget, and by pumping the carbon dioxide into the ground to bring lucrative oil back to the surface, the project generates revenue to defray its capital costs. Nearby, the start-up NET Power has built an even more advanced pilot plant in which supercritical carbon dioxide derived from burning natural gas drives a turbine, making it simple to capture the resulting carbon emissions. Still, much more progress needs to be made, both on the technology to capture carbon as well as to invent valuable uses for it. If plants can make money by selling carbon dioxide to make cement for buildings or carbon fiber for planes and cars, for example, then the attractiveness of zero-carbon fossil electricity could skyrocket.35
With a strong foundation of flexible-base power in place, the quantities of solar power and energy storage required to achieve deep decarbonization become much more tractable. Continued cost declines for both solar and storage will nonetheless be important to make their deployment cost-effective. Storage can improve the economics of solar power.36 Therefore, it makes the most sense to talk about solar generation and storage costs in the same breath rather than separately. One study found that if the costs of both solar and storage were to plunge together, the share of solar in the American West could triple. This intuitively makes sense—solar paired with storage looks to the grid like a really flexible power plant, so their combined cost would drive the optimal level of deployment. Don’t forget about flexible base, though; the same study found that even with rock-bottom solar and storage prices, the most cost-effective power mix included a 43 percent share of supply from flexible base.37
The lesson here is that deep decarbonization of the power sector—a prerequisite for limiting climate change—will require a diverse cast of characters. Both flexible-base power and fast-acting resources, including those that store energy, are jointly needed to enable solar power to shine. Alas, deep decarbonization is not one of the goals of the free market. As a result, it has become increasingly difficult for all three categories of zero-carbon power resources to coexist in the rough-and-tumble of power markets.
From Capacity to Capability
Another aspect of systemic innovation, in addition to designing the power mix of the future, is conceiving the markets that will enable that mix to emerge. Chapter 3 recounted the ways that the rise of solar has wrought havoc on power markets around the world, from Chile to Germany. Surplus solar power is forcing the price commanded for the resulting electricity below zero and driving other kinds of power plants out of the market. In particular, flexible-base power plants are struggling to stay in business, even though they are crucial to enabling high penetration of the renewable energy that is slashing their bottom lines right now.
For example, nuclear power plants from Germany to the United States are shutting down, largely because they can no longer cover their costs. The U.S. nuclear reactor fleet—which generates a fifth of the country’s electricity and most of its zero-carbon power—is in danger of losing half its reactors to early shutdowns by 2030.38 Nuclear’s economic woes stem from two main causes (other than project mismanagement): cheap natural gas and a surge in solar and wind power. Both trends have upended the way that electricity markets have historically kept nuclear plants in business.
In the old paradigm of a power market, every power plant would provide a bid for how much power it could produce and the price at which it was willing to sell it to the grid. Nuclear plants would offer to sell power at a low cost because once a plant is up and running, spitting out the next kilowatt-hour of energy does not cost much. Fortunately for those plants, the market would accept bids in ascending order to meet all the customer demand. So, once bids from the low-cost plants, such as nuclear reactors, were accepted, the market would have to accept higher-cost bids, such as from natural gas turbines that would charge an arm and a leg to burn expensive fuel and boost output quickly when demand surged. Markets also paid every power plant the same, “market-clearing” price—that is, the price charged for the last bid accepted (that of the expensive natural gas turbine). This practice was a relief for the nuclear reactors because, despite the low cost of producing power, the plants relied on high ongoing revenues to pay off the hugely expensive construction costs of the plant.
Together, cheap renewable energy and natural gas have turned that old market paradigm on its head. As the penetration of solar and wind power rises, these generators are happy to offer to sell their power at zero cost to the grid because they have zero fuel costs. Their influence, along with that of cheap natural gas, drags down the market-clearing price. In other words, solar and wind generators compete with nuclear power to have their low-cost bids accepted by the market. Whoever wins ends up getting paid only a pittance. And nuclear plants that can no longer pay off their capital costs go bust.
The same thing would happen to the economics of solar and wind generators, except that they are protected by policy or contract structures. Public incentives, such as the German feed-in tariff, supplement the income that renewables ordinarily would make from depressed market prices. And because most solar power is contracted through long-term power purchase agreements (PPAs) with a locked-in price, solar owners are guaranteed that their costs will be covered even as the market price craters. Some places, such as the state of New York, have opted to provide incentive payments to nuclear plants to similarly insulate them from an imploding market.39 But in most other U.S. markets, nuclear is left out in the cold as renewables thrive.
Today’s markets thus have a “missing money” problem: they fail to pay enough to flexible-base plants, such as nuclear reactors and fossil-fueled plants (whose emissions, ultimately, need to be captured and stored), for investors to want to build more. This state of affairs is untenable if deep decarbonization is to be achieved.
Some jurisdictions have tried to fill the missing money gap; their efforts fall into three categories. First, California (among others) obligates the utilities that transmit and distribute power to sign long-term contracts with reliable generators to guarantee that they will have enough power for forecast customer demand—plus a 15 percent safety margin. This way, investors in power plants have some long-term security that the rug won’t be pulled out from under them as rising solar output reduces market prices. Second, most of the states on the U.S. East Coast, as well as several European countries like the United Kingdom, have created separate “capacity” markets, which pay reliable generators to sit around until the grid calls on them urgently for support.
The third category is exemplified by Texas, which is known for going it alone. A paragon of free-market economic purity, Texas has preserved an “energy-only” wholesale market that works just as in the past, except with an extremely high cap on the instantaneous price for a kilowatt-hour of electricity. Under this approach, if power supplies are really scarce, a power plant can save the day by selling its wares for $9,000/kWh. That mouthwatering possibility, even if it happens only once every year or even less frequently, is a strong incentive for investors to build flexible power plants. But, in most places, the prospect of astronomical power prices is a political nonstarter—and some scholars argue that the missing money problem would still persist.40
Each of these approaches has its own die-hard adherents. In Europe, an ongoing effort to create a unified, continentwide power market faces hurdles owing to the twenty-eight different capacity market schemes created by the member-states of the European Union.41 Some members, including Germany, want to abolish capacity markets altogether. Others, including France, which does not share Germany’s obsession with shutting down nuclear plants, are not so sure. Many argue that, by paying reliable plants to sit around rather than actually producing power, capacity markets raise customer prices. Just as many others counter that this approach is a sort of insurance that is well worth the investment, lest rolling blackouts and surging prices cost customers even more dearly.
Setting this stale debate aside, other, innovative options could modernize power markets, accommodate a rising share of renewable energy, and fund a strong foundation of flexible-base power. The first priority is minimizing the amount of expensive reserves needed to accommodate renewable energy unpredictability. Better weather forecasting technology is emerging that can help by enabling grid operators to predict more accurately what solar and wind output will be like hours or days in advance and calling in compensating resources accordingly.42 The advent of “deep learning”—the artificial intelligence algorithms that run your Alexa device at home and learn from past experience— could make forecasts of solar production even more accurate and precise.43
In addition, markets should operate on a quicker cadence, reducing from an hour to five minutes or less the time interval between successive decisions on which generators to dispatch and how much to pay them. More powerful computers and smarter grids make this overdue change possible.44 This would enable grid operators to dispatch other generators to react to renewable energy volatility most efficiently, rather than relying on the crutch of overbuilt reserve capacity.45
Another important step is to retool power markets so that they value the capability to meet the grid’s needs, not just the nameplate capacity of how much power a generator can provide. An essential capability is the provision of inertia. Recall from chapter 3 that conventional power generators give the grid inertia, so if one plant goes down, the synchronized rotation of other generators prevents the grid’s frequency from plunging for long enough to allow alternative supply sources to ramp up. But solar panels do not produce electricity through physical rotational motion, and thus do not provide any inertia to the grid. Inverters that connect solar to the grid can be programmed to provide synthetic inertia, however, that recreates the effect of the real thing. A study of the western United States found solar power would achieve much higher penetration if all utility-scale solar plants had smart inverters that provided synthetic inertia.46
In fact, solar plants can do even better than conventional power plants when it comes to supporting the grid and ensuring that the power system operates within narrow frequency and voltage bands.47 By delivering such ancillary services, solar power could be part of the solution rather than the problem. In 2017, First Solar demonstrated just how useful a utility-scale solar plant could be to the grid. In collaboration with California’s grid operator, a 300-megawatt (MW) First Solar plant proved that it could beat conventional natural gas plants at rapidly boosting the grid’s frequency level.48 Another plant in Arizona was even able to support the grid in the middle of the night, when its solar panels produced no power but its sophisticated power electronics propped up the voltage on a transmission line after an unplanned shutdown of an upstream nuclear reactor.49 California is already starting to compensate solar plants for the advanced capabilities that they offer. If other states and countries do the same, developers might follow suit and build advanced solar plants that can stabilize the grid.
In addition to the market tweaks described above, even more fundamental changes to the design of electricity markets could help the power system accommodate expanding use of intermittent renewable energy. For example, some scholars have suggested splitting wholesale energy markets into two in order to insulate flexible-base and renewable resources from destructive competition. The first market would be for on-demand, or firm, power from plants whose output can be reliably controlled. The other market would be for variable power from unpredictable generators, such as solar and wind, which cannot guarantee their output.50 Prominent energy analyst Michael Liebreich argues that the difference in prices between the two markets, which he dubs the “Firm Spread,” will rise as value deflation accompanies the rise of variable solar and wind power. And that spread will attract reliable sources of firm generation capacity, as well as innovation to develop such new, flexible resources as virtual power plants or storage technologies.51
Ideas like that one are still very much on the drawing board. But that is the sort of innovative market design that might be required to ensure that a diverse mix of zero-carbon power resources coexists. Indeed, the power sector is supposed to be the easy one to decarbonize. The rest of the economy, starting with transportation, presents even thornier challenges.
Batteries on the Go
The most obvious way to extend decarbonization from the power sector to the transportation sector is through vehicle electrification. Seduced by Elon Musk’s sleek creations, masses of customers would buy EVs, plug them into their garage outlet, and replace oil with electricity produced by zero-carbon sources. Simple, right?
Probably not. Even if customers were to flock to EVs—which is still very uncertain—it is unclear whether the grid would be able to cope with them. Level II chargers, which can recharge an EV in a few hours, add the equivalent of an entire household’s power demand to a neighborhood distribution circuit. These circuits are not sized to handle many EVs, since they normally serve only five to ten homes. Even worse, charging a Tesla at one of the company’s Supercharger stations suddenly sucks as much power from the grid as two hundred window air-conditioning units running all at once. So, utilities are anxiously eyeing massively expensive upgrades across the distribution grid if EV adoption really takes off.52
The story would be different if EVs could support, rather than strain, the grid. After all, from the grid’s point of view, an EV is just a battery on the go. If EV batteries could double as an energy storage resource for the power grid, the 50 million EVs that Bloomberg projects will be sold globally in 2040 could be put to work smoothing the volatile output from solar power.53
Indeed, linking the transportation and power sectors could add even more flexibility to integrate a high penetration of solar power, although doing so won’t be easy. In the near term, as smart grids learn how to talk to EVs, they might orchestrate small changes in how fast an EV charges up while plugged in. This cooperation could provide ancillary services for maintaining the grid’s voltage and frequency levels without an EV driver ever noticing the difference. In the longer term, as more EVs hit the roads and grid operators become more sophisticated, the grid could actually harness the full capability of EV batteries to absorb or supply energy as needed. EVs, acting in concert with other grid resources, such as stationary batteries and power plants, could store solar power and discharge it when customer demand peaks.54
Transportation is not the only energy sector that could be linked to the power sector to provide flexibility. Linking the heat and power sectors could be an equally important way to enable a high penetration of solar power. Such a link would not be new. Existing cogeneration plants, which produce both power and heat, can serve densely populated communities, as well as such industrial facilities as chemical plants, refineries, and paper mills that require both electricity and heat. Expanding cogeneration is one way to harness sunlight to meet demand for heat. Although most cogeneration today relies on burning fossil fuels, it could be powered by solar thermal collectors. Indeed, pilot projects from Denmark to Saudi Arabia have demonstrated the viability of using solar thermal to drive the cogeneration of both power and heat. In those projects, heat from the cogeneration facilities was piped into district-heating networks, which connect a community’s homes and buildings and can more efficiently provide heat than individual boilers.55
In addition to combining the production of heat and power through cogeneration, it is possible to manage the demand for heat in ways that would smooth out the amount of electricity that utilities deliver to customers in the course of a day. New electric heat pumps and electric water heaters are highly efficient at space and water heating, either for individual homes or for district heating networks. A smart grid connected to such devices could switch on heaters when the electricity supply is high, essentially pre-heating a room or hot-water tank in anticipation of need, rather than waiting for customers to call for heat only later, when solar energy is no longer available.56
In effect, just as was the case with linking the transportation and power sectors, linking the heat and power sectors could provide another big battery to store intermittent solar power in the form of hot air and water. Indeed, integrating all three of these sectors would maximize the flexibility of the combined power-heat-transportation system.57 Electrification—of both vehicles and heating appliances—is one way to accomplish this. And if solar-driven water splitting technology, discussed in chapter 7, were to become commercially viable, then solar could drive a hydrogen economy that would also link the transportation and heating sectors.
Aware of such possibilities, just before President Barack Obama left office, his administration released an ambitious “United States Mid-Century Strategy for Deep Decarbonization” road map. It projects that for the United States to reduce its economywide carbon emissions by 80 percent by 2050, 60 percent of the miles driven by vehicles will need to be fueled by either electricity or hydrogen, and electricity must supply the majority of space and water heating.58
Chapter 8 introduced the concept of how linkages between the electricity grids of different regions to form a supergrid could raise the potential penetration of solar power. This chapter added others, between the power, transportation, and heating sectors. These linkages all add flexibility to the overall energy system, making it possible to run an economy off volatile solar energy. But the potential benefits of interconnectivity don’t end there; interactions with processes outside of today’s energy sector offer additional ways for the electricity sector to accommodate intermittency.
Beyond Energy
On July 31, 2012, an unseasonably dry summer day, the biggest blackout in human history hit India. Altogether, 700 million people lost access to power (adding that figure to the numbers of people without any access to begin with meant that more than 1 billion Indians went without electricity that day). The culprit? Grid-connected irrigation pumps.
Delayed monsoon rains had driven millions of farmers to set their pumps on overdrive to suck up groundwater from deep underground to irrigate their crops. The combination of the spike in electricity demand and the dilapidated Indian electricity grid was too much for the system to handle. This episode was a stark reminder that energy, water, and food systems are inextricably intertwined, and the needs of the food and water sectors could increase demands on the energy sector. Intelligent management of those connections, however, can increase the flexible operation of each sector and ease the strains they put on one another. Indeed, done right, increasing the links between energy, water, and food offers yet another way to accommodate a rising share of volatile solar energy and put that energy to good use.
Start with water. Countries around the world will increasingly face water scarcity as populations grow and the climate changes. Already, the production and transportation of freshwater require massive energy consumption. Nowhere is this more true than the parched Middle East. Much of the region’s freshwater supply comes from desalination of seawater—a technology so energy-intensive that it consumes between one-tenth and one-third of the electricity produced in countries in the Persian Gulf.59
Those desalination plants could provide flexibility for the grid to accommodate a high share of solar power. By adjusting their output, desalination plants can modulate their demand for electricity, operating more when the sun is shining and less when it is not, and therefore making use of excess daytime solar capacity. There are economic limits to how flexibly they can run—if they operate below full output for too long, they will struggle to earn enough revenue from water sales to cover their capital costs. But, they could certainly be an additional puzzle piece in constructing a flexible, solar-powered grid.60 The biggest benefit could be cheap water. Recognizing this, Saudi Arabia wants to slash the cost of its water by over 80 percent by investing heavily in solar-powered desalination.61
New desalination technologies are on the horizon that could also take advantage of the waste heat from a concentrated solar power (CSP) plant, improving its economics compared with a CSP plant producing only electricity. The most popular desalination technology today—reverse osmosis, which forces salty water through filtration membranes—runs on electricity. But multiple-effect distillation desalination, in which heat is used to vaporize water in multiple stages and leave behind the salt, could become cost-competitive if the technology advances. Combining this technology with CSP could make fresh water even cheaper in the thirsty Middle East.62
What about food? The 2012 blackout that occurred in India when the agricultural sector’s pumping of water overwhelmed the grid highlights the way a poorly managed linkage between the power and agricultural sectors can lead to disaster. In this case, it might actually make more sense to delink agricultural demand for irrigation from India’s overstretched power grid.
Irrigation pumping is an ideal application for distributed solar power because it does not require a highly reliable and constant power supply and can instead tolerate volatile solar output. If their irrigation pumps were powered by solar panels, farmers would not need to connect them to the grid.
The use of solar would have a couple of major benefits. First, fewer connected pumps would lower demand on the strained power grid. Second, solar-powered irrigation pumps could limit wasteful pumping practices. Farmers in India receive subsidized electricity from the grid, perversely incentivizing them to run their pumps more often and deplete groundwater supplies. Indeed, India is the largest user of groundwater for agricultural purposes in the world. If a solar irrigation pump were designed to pump only a sustainable amount of water, it could better preserve groundwater supplies. (If solar costs continue to fall, however, oversized solar irrigation systems might also enable wasteful pumping.) The Indian government has embarked on an ambitious program to replace 26 million groundwater irrigation pumps with solar pumps.63
A pilot project planned in Karnataka, in the south of India, aims to harness sunlight to provide electricity, food, and water to nearby communities. The first step will be to concentrate solar radiation with mirrors to generate steam. That steam will then drive a turbine to generate electricity, and the waste heat will be used to distill water and provide refrigeration to preserve food.64 In a sense, the distilled water and preserved food are storage technologies, converting ephemeral sunlight into a more permanent form that can be consumed when needed.
Neither of those storage technologies is anywhere near as flashy as the battery packs on the Desperate Housewives set under Elon Musk’s solar roofs. But they are nonetheless important pieces for solving the puzzle of how the world can harness sunlight to meet not only its energy needs, but also other basic needs, such as food and water.