There is a story, possibly apocryphal, of the distinguished British biologist J. B. S. Haldane, who found himself in the company of a group of theologians. On being asked what one could conclude as to the nature of the Creator from a study of his creation, Haldane is said to have answered, “An inordinate fondness for beetles.”
—J. E. Hutchinson, “Homage to Santa Rosalia or Why Are There So Many Kinds of Animals?”
Most people are interested only in our own phylum Chordata, which includes vertebrates. They don’t know or care about clams, snails, and “bugs” (or they consider them disgusting and don’t want to know). Typically, people call most members of the phylum Arthropoda (including not only insects but also spiders, scorpions, “pillbugs,” centipedes, millipedes, lice, ticks, and many other unrelated groups) “bugs” and think of most marine invertebrates that are worth eating as “shellfish.” Nevertheless, the invertebrates make up more than 99 percent of all living animals on earth. In fact, insects alone outnumber all other groups of organisms in total diversity, and among insects, beetles are more diverse with more species than any other group of animals. Despite the lack of public familiarity, invertebrates are not only the most diverse animals alive today, but they also include the best-fossilized groups by far. We will devote the remaining chapters of this book to looking at more familiar and more popular examples from birds, mammals, and reptiles, but we cannot neglect the excellent fossil record of transitions within the invertebrates. The invertebrates may not be as cute and cuddly as mammals or birds, but they show us far more about evolution than the much less complete fossil record of vertebrates.
Unknown to Darwin, uninterrupted sedimentation does occur in the open ocean, especially on aseismic ridges and plateaux. These areas experience a continuous rain of particles to the sea bed, and are among the most geologically quiescent places on Earth. A steady build-up of sediment is the result…. The sediments in question are composed mainly of the shells of microscopic plankton such as foraminifera, radiolaria, diatoms and coccolithophorids. Large numbers of individuals can easily be extracted. Their evolution can be followed through geological time, simply by comparing one closely-spaced sample with the next. This reveals morphologically isolated and continuous lineages which it is reasonable to infer represent lines of genetic descent. These lineages sometimes split from one another, and often evolve gradually over vast periods of time, or become extinct…. Does the fossil record provide a true and accurate record of first and last occurrences of species? Emphatically, the answer is yes! Microfossils are used routinely for biostratigraphic correlation by thousands of specialists the world over. This would not be possible unless the sediment record was good and reliable. We now know (within fairly precise limits) when hundreds of species of mineralizing plankton arose and became extinct, through a history that spans over a hundred million years.
—Paul Pearson, “The Glorious Fossil Record”
Although the evolution of dinosaurs and humans is a far more glamorous subject, by far the best fossil record is found in the microscopic fossils left behind by single-celled organisms in the deep sea. These protistans occur by the trillions in many larger oceanic water masses, and their shells literally carpet the shallow seafloor with hundreds to thousands of individual specimens in a cubic centimeter of sediment (fig. 8.1). Their density can exceed a million specimens per cubic meter of sediment and weigh up to 10 grams per cubic meter. Most of the open ocean floor shallower than 3,000 m is completely covered by “calcareous ooze” composed of the skeletons of microfossils made of calcium carbonate. The sand of many tropical beaches is composed almost entirely of the skeletons of microfossils. In a typical sample of tropical marine sediment, there may be 60 to 70 species. In some groups, such as the foraminifera, there are over 3,600 described genera and perhaps 60,000 species, making them more diverse than any other group of marine animals or plants.

FIGURE 8.1. A typical assortment of planktonic microfossils from a deep-sea core. Each is about the size of a pinhead or smaller, and many thousands are found in every cubic meter of sediment. They include the foraminifera (larger bubble-shaped shells) and the radiolaria (smaller porous conical shells), along with long, spikelike sponge spicules. (Photo courtesy Scripps Institution of Oceanography)
In addition to their great abundance and diversity, microfossils are ideal for evolutionary studies for several other reasons. Cores of the sediments covering the deep-sea bottom have been taken by rotary drilling and by plunging a long tube into the sea bottom (“piston coring”), and both retrieve an almost continuous record of marine sedimentation over that part of the ocean floor. Some cores span many millions of years with no breaks or gaps whatsoever. These cores can be precisely dated by methods such as stable isotope analysis and magnetic stratigraphy, as well as with the biostratigraphy of the microfossil groups themselves. Thus we can trace the history of many microfossil lineages through many millions of years over a single spot in the world, something that is impossible with the much less complete record of shallow marine invertebrates or land vertebrates. Finally, the biogeography of microfossils is relatively simple. Most are confined to a few water masses where the ocean waters are of a given temperature, and these species range over that entire water mass (Prothero and Lazarus 1980). Thus a few cores from an area representing a single water mass will sample all the populations in that water mass, and there will be no small “peripheral isolate” populations that could be missed. As a result, Prothero and Lazarus (1980) showed that if we have cores representing most of the world’s major water masses in a given time interval, we can look at a lineage or group and see practically all there is to see about the evolution of their skeletons. Prothero and Lazarus (1980) argued that microfossils are our best “laboratory animal” or “fruit fly” to study evolution in the fossil record.
Naturally, there are a few drawbacks to using microfossils to study evolution. The biggest problem is that we still know relatively little about the biology of the living relatives. Some are difficult to keep alive in the laboratory once they have been caught. Researchers have studied the few species that can be cultured only over short intervals of time. Besides, the most valuable information about their biology relates to how they live in the open ocean, which is hard to simulate in a lab. In addition, microfossils have relatively simple skeletons, without the many levels of anatomical detail that you find in many macroinvertebrates or vertebrates. There is good evidence that some forms have evolved more than once through convergent evolution (Cifelli 1969). In other cases, it is not clear that the skeletal shape is that well constrained during the life of the organism. Finally, what we do know of the biology of these organisms suggests that many of them are asexual through at least part of their life histories and reproduce by cloning, especially when they are trying to multiply quickly to exploit an abundant food resource. Others may hybridize across lineages (Goll 1976), ending the reproductive isolation of species that characterizes multicellular animals. Thus we cannot always be sure that the rules about speciation and evolution developed for sexually reproducing multicellular animals and plants (such as Mayr’s allopatric speciation model discussed earlier) can be applied to partially asexual or hybridizing microfossils.
But every research problem has its strengths and limitations, and the strengths of micropaleontology are so enormous that it has proven one of the most fruitful areas of research in all the geologic sciences. Hundreds of micropaleontologists work for oil companies, helping to precisely date and correlate the formations that they drill through to find oil or helping to determine the depth of the water in which ancient oceanic sediment was deposited. Other micropaleontologists work mostly as marine geologists and paleoclimatologists, using microfossils to determine how oceanic currents and climate have changed through time. A small number of micropaleontologists study the biology and shell chemistry of these organisms, and even a smaller number are interested in using microfossils as exemplars of evolution. Despite this trend, however, there are hundreds of well-documented examples of evolution in the microfossil record, of which we will have space to mention only a few.
Although there are many types of microfossils that could be studied (see Prothero 2013a: chap. 12), the most important ones include just a few groups. Two are animallike protistans related to the amoeba, with its oozing, flowing protoplasm—but unlike an amoeba, they have internal mineralized shells. The most diverse and most widely studied are the Foraminifera (fig. 8.2A) or “forams” for short, which secrete skeletons of calcium carbonate (the mineral calcite). Most forams live on or in the sea bottom (benthic), but one family, the Globigerinidae, are tiny and buoyant and make up a major part of the marine plankton. The second group of amoeba-like plankton is the Radiolaria (or “rads” in the trade), which secrete skeletons of opaline silica instead of calcium carbonate. These delicate porous glassy skeletons have been compared to miniature Christmas ornaments in their beauty and symmetry (fig. 8.2B).

FIGURE 8.2. Examples of common groups of planktonic microfossils. (A) A foraminiferan, showing the bubble-shaped chambers made of calcite surrounded by long fingers of protoplasm known as pseudopodia. (Courtesy J. Kennett) (B) A radiolarian, with its characteristic porous spiny shell made of silica. (From Haq and Boersma 1978) (C) A diatom, with its perforated petri dish–shaped shells made of silica. (Courtesy J. Barron) (D) A coccolithophorid alga, surrounded by multiple plates (coccoliths) made of calcite. (Courtesy W. Siesser)
The other important groups of microfossils are actually planktonic plants, members of the division Chrysophyta, or the golden-brown algae. They include the diatoms, which secrete their skeletons out of silica and are found in marine and fresh waters all over the world (fig. 8.2C), and the coccolithophorids, which secrete hundreds of miniscule (a few microns in diameter) button-shaped plates over their spherical cells (fig. 8.2D). These phytoplankton (planktonic algae) are the base of the entire food chain in the world’s oceans, and all other organisms (from the foraminifera and radiolaria to megascopic predators like crustaceans to fish all the way up to whales) feed directly or indirectly on them. In addition, phytoplankton are the single largest producer of the oxygen we breathe (much more important oxygen producers than land plants), and in many places in the ocean they are so abundant that they pave the sea bottom with trillions of their shells. In fact, the rock known as chalk is actually made of millions of skeletons of coccolithophorids and a few foraminifera. Phytoplankton are so important to life on this planet that any major crisis in their evolution has caused mass extinctions all the way up the food chain. To a great extent, none of us would be here without the oxygen that phytoplankton release, and the food that they provide for the life of the sea.
Let us look at some of many examples of evolution in the well-studied forams first. One of the classic examples of long-term evolution in the foraminifera is provided by a group known as the Fusulinidae (fig. 8.3). Fusulinids were benthic (bottom-dwelling) foraminifera, so they were not constrained to be tiny and float in the plankton. Instead, they secreted shells that ranged from the size of a grain of rice up to 5 centimeters (2 inches) long, which is enormous for a single-celled organism. Like many modern large benthic foraminifera, fusulinids probably harbored symbiotic algae within their tissues to enable them to grow so large. That idea is confirmed by the fact that fusulinids lived in enormous numbers on shallow sea bottom (probably shallow enough for light penetration) during the late Paleozoic (Mississippian to Permian periods, 355–255 million years ago). In many places, huge volumes of limestone are made of nothing but fusulinids, comprising trillions of individuals (fig. 8.3). Then, at the peak of their success, they were wiped out by the greatest mass extinction event in earth history, the Permian catastrophe, which extinguished 95 percent of the marine species on the planet. Whatever the cause of this great mass extinction, the fusulinids were one of the most conspicuous victims.

FIGURE 8.3. The bottom-dwelling (benthic) foraminifera known as fusulinids were about the size and shape of a grain of rice and extraordinarily abundant in late Paleozoic limestones, where they may number in the trillions and make up entire rock units. (Photography by W. Hamilton, courtesy U.S. Geological Survey)
The fusulinid shell is shaped like a spindle or a grain of rice (figs. 8.3 and 8.4). As the shell grew, more and more shell layers were added, spiraling around the long axis of the spindle, so that when you cut it across the middle, you typically see a spiral pattern. In cross section, you can see that the spiral layers are supported by a dense network of smaller walls and chambers, with an intricate, complex structure. These complex wall structures make each genus and species of fusulinid distinct and easy to recognize for the specialist. Fusulinids evolved rapidly through the late Paleozoic (fig. 8.4) from simple forms with only a few chambers like Millerella from the Late Mississippian and earliest Pennsylvanian, to a variety of different lineages that become larger with more and more complex wall structure and interesting variations on the spindle-like symmetry. The enormous variations within this basic body form are apparent even to the nonspecialist and provide a dramatic example of evolution within a single lineage. To the specialist, the different species are so distinctive, and their fossils are so widespread and abundant in late Paleozoic limestones, that they are the principal method of dating rocks of late Paleozoic age. If you want to know the age of any marine limestone from the Pennsylvanian or Permian, ask a fusulinid expert to look at it, and you will get the most precise estimate possible.

FIGURE 8.4. (A) Fusulinids evolved very rapidly during the late Paleozoic (Pennsylvanian and Permian) by developing increasingly more complex chambers and wall structure, and a variety of shapes based on the fundamental plan of a spiral shaped like a spindle. (B) Some photos of fusulinids at natural size. (Modified from Boardman et al. 1987; courtesy Blackwell Scientific Publications)
There are many other examples of dramatic transformations in the forams that could be shown. For example, in the Pliocene, one of the common planktonic forams is Globigerinoides sacculifer (Kennett and Srinivasan 1983), which has a shell shaped like a series of porous oblong bubbles clustered together in a spiral arrangement (fig. 8.5A). Through the many cores that sample the Pliocene oceans we can find more and more specimens that develop these long slender fingerlike extensions all over the final few chambers. As you move up the cores, these little “fingers” become longer and more common. These creatures are so distinct from the ancestral lineage that they branched away from that they are given their own species: Globigerinoides fistulosus (they do indeed look like little fists). Another common trend is the gradual evolution of foraminifera with flatter chambers and keels along the edges from species with more primitive bubble-shaped chambers. These trends can be seen in the evolution of keeled Morozovella from Praemurica in the late Paleocene (fig. 8.5B), in keeled forms of Globoconella in the early Miocene (fig. 8.5C), and in keeled Fohsella, also during the early Miocene (fig. 8.5D).

FIGURE 8.5. Evolutionary sequences in some of the planktonic foraminifera. (A) In the Pliocene and Pleistocene, the fingerlike projections of Globigerinoides fistulosus evolved from the smooth-shelled Globigerinoides sacculifer. (B) The evolution of Praemurica (top) to the keeled Morozovella (bottom) that occurred between 63 and 59 million years ago. (C) The evolution of Globoconella in the early Miocene (20–18 million years ago), from the unkeeled forms at the top to the keeled Globoconella conoidea at the bottom, which vanished about 6 million years ago. (D) A similar trend in the genus Fohsella, from the ancestral unkeeled forms at the top to the highly keeled species at the bottom. (Photos in (A) courtesy J. Kennett; (B–D) courtesy R. Norris)
Let us look at the other amoeba-like group, the radiolarians. As we mentioned already, this group is much like the forams, only their skeletons are made of opaline silica (fig. 8.2B). Unlike forams, which are both benthic and planktonic, all radiolaria are planktonic, so their evolution and ecology closely mirrors the change in water temperature and chemistry where they live and grow. Most of the time, they flourish only in places where nutrients are brought up from the deep ocean to the surface. To the radiolarian, the scarcest and most important nutrient is silica itself, which is depleted in normal surface seawater. When silica is carried up from the deep by upwelling ocean currents, radiolaria and diatoms bloom in enormous numbers and consume almost all of it immediately. After they die, their delicate skeletons rain down on the ocean floor by the millions, so any ocean sediment in areas of upwelling (typically in the boundary currents between water masses) is full of siliceous plankton such as radiolaria and diatoms.
When I was a graduate student at Columbia University and at the American Museum of Natural History in the late 1970s and early 1980s, I decided to learn about micropaleontology to balance out my education in fossil vertebrates and invertebrates. Affiliated with Columbia is Lamont-Doherty Geological Observatory (now Lamont-Doherty Earth Observatory), one of the foremost geologic research institutions in the world. Lamont was the place that led the revolution in geology known as plate tectonics in the 1960s. I rode the shuttle bus up the Hudson River to Lamont so that I could take classes from the giants of plate tectonics, paleomagnetism, and seismology. Lamont has long been one of the pioneers in oceanography and marine geology, with a collection of deep-sea cores from the oceans of the world that is second to none. While at Lamont, I spent most of my time in the core laboratory, where I got to examine microfossils by the thousands and learned about foraminifera from Tsunemasa Saito, radiolaria from Jim Hays, and diatoms from Lloyd Burckle. Soon I was working on a research project with my fellow graduate student Dave Lazarus, counting and measuring hundreds of specimens on slides and trying to decipher the evolutionary patterns in a group of radiolaria known as Pterocanium (Lazarus et al. 1985). These cute little “Christmas ornaments” were shaped like a lacy bell (the thorax) with a knob (the cephalis) and a spike on top, and three long spines sprouting out from the open base (fig. 8.6). From the ancestral form Pterocanium charybdeum allium (so named by Dave because the thorax was shaped like a clove of garlic, allium in Latin) that lived 7 million years ago, we documented a complex pattern of divergence among different shapes. Some developed larger pores and more robust spines that flared out from the base and lost the distinct “knob” of the cephalis at the top (Pterocanium audax). Another lineage developed a more cylindrical, boxy shape with distinct “shoulders” (Pterocanium prismatium, an important index fossil for the Pliocene). Another lineage developed huge flaring spines and shrank the size of the thorax to a little ball (Pterocanium korotnevi). Two lineages that had been distinct for millions of years (Pterocanium praetextum and Pterocanium charybdeum trilobum) appeared to hybridize during the late Pleistocene. The diagram in figure 8.6 does not capture how the change can be traced gradually centimeter by centimeter through many different cores, so we could see every transitional step between one extreme shell shape and another. However, it was possible to measure many different features, such as the length of the thorax, and see the gradual shift of sizes in each population spanning 7 million years.

FIGURE 8.6. Evolutionary patterns in the radiolarian Pterocanium from the late Cenozoic. Solid bars show the time distribution of the main lineages, while gray zones show intervals of intergradation and possible hybridization of lineages. Time scale along left indicates millions of years before present (Ma). Scale bars = 10 micrometers. 1, Pterocanium korotnevi; 2, Pterocanium praetextum; 31, Pterocanium charybdeum allium; 32, Pterocanium charybdeum charybdeum; 33, Pterocanium charybdeum trilobum; 4, Pterocanium prismatium; 5, Pterocanium audax. (From Lazarus et al. 1985: fig. 21; used by permission of the Paleontological Society)
This is just one example of evolutionary changes among the radiolaria, and there are hundreds more that could be cited. In fact, radiolaria are still not that well studied (especially compared to forams), so there could be hundreds more as yet unknown. Let us look at just one more classic example, probably the most extreme change in morphology ever documented in the fossil record. If you look at samples of microfossils from the middle Eocene (50 million years ago), you will find distinctive spongy ball-shaped radiolarians known as Lithocyclia ocellus (fig. 8.7). As you trace the spongy balls up through the sediments spanning millions of years, you see them gradually lose their spongy outer layers and develop into a small nucleus with four spongy arms (Lithocyclia aristotelis), then three arms (Lithocyclia angusta), and finally two arms forming a spindle-like shape (Cannartus tubarius). The Cannartus lineage then gradually develops a “waist” on the central sphere, then the arms get shorter and thicker, and finally, they split into two lineages: Cannartus peterssoni-Ommatartus hughesi, which evolves into a form with two arms with multiple spongy layers, and Ommatartus, which develops shorter arms and a fatter central sphere. If you look at the two extremes (a spongy sphere turning into a spindle-shaped shell with multiple caps), you could never imagine that they are closely related—yet I have looked at the slides from those cores and seen the gradual transition from one extreme to the other with my own eyes.

FIGURE 8.7. Evolutionary transformation in the cannartid-ommatartid lineage of radiolaria over the past 50 million years, from spongy balls to four- and then three-armed and finally two-armed bipolar structures, with further variations in the spongy caps later in their evolution. Taxa are as follows: 26, Lithocyclia ocellus; 27, Lithocyclia aristotelis; 28, Lithocyclia angusta; 30, Cannartus tubarius; 31, Cannartus violina; 32, Cannartus mammiferus; 33, Cannartus laticonus; 34, Cannartus petterssoni; 35, Ommatartus hughesi; 36, Ommatartus antepenultimus; 37, Ommatartus penultimus; 38, Ommatartus avitus; 39, Ommatartus tetrathalamus. (Modified from Haq and Boersma 1978)
There are multiple studies on evolutionary patterns and transitions in other microfossils, including the diatoms and coccolithophorids (see Lazarus [1983] and the articles in Paleobiology, volume 9, number 4, fall 1983 for examples). For space reasons, however, we will leave the extraordinary record of microfossils and look at the patterns in the more easily studied macroinvertebrates.
Why don’t paleontologists bother to popularize the detailed lineages and species-to-species transitions? Because it is thought to be unnecessary detail…. Paleontologists clearly consider the occurrence of evolution to be a settled question, so obvious as to be beyond rational dispute, so, they think, why waste valuable textbook space on such tedious detail?
If you find it difficult to relate to tiny fossils that can only be studied with expensive microscopes, you can go right out to your local fossiliferous outcrop and study patterns of evolution there. Take, for example, the famous cliffs along the shores of Chesapeake Bay (fig. 3.2A and B) that are made of solid shells of mollusks from the Miocene (about 18–5 million years ago). Some of the most common and distinctive fossils are the large scallops known as Chesapecten. One of these species, Chesapecten jeffersoni, is the state fossil of Virginia. As numerous studies have shown (Ward and Blackwelder 1975; Miyazaki and Mickevich 1982; Kelley 1983), the shells of these scallops change continually through time. The earliest forms are known as Chesapecten coccymelus (fig. 8.8); they are abundant in Zone 10 of the middle Miocene Calvert Formation. As you pass up from the Calvert Formation to the Choptank Formation, there are specimens of Chesapecten nefrens, which have shells that are longer from front to back than they are high from hinge to opening; this trend continues through the series. As you move up through the series, the number of ribs decreases as well, except in Chesapecten middlesexensis, which reverses the trend and develops more ribs. Many more subtle differences in the shells can be detected (Ward and Blackwelder 1975), so many that any competent paleontologist can tell the species apart easily and decide what time in the Miocene or Pliocene is represented just by the scallops.
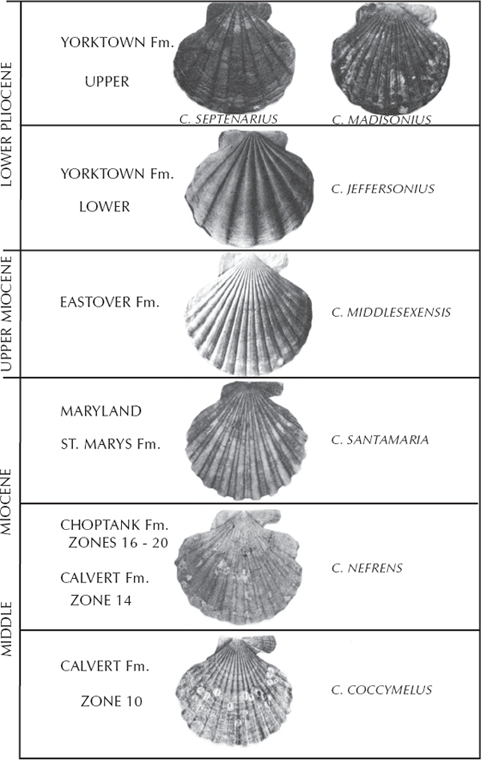
FIGURE 8.8. Evolution of the scallop Chesapecten during the Miocene and Pliocene, as preserved along the Calvert Cliffs in Chesapeake Bay. (After Miyazaki and Mickevich 1982: plate 1; originally based on Ward and Blackwelder 1975; used by permission of Plenum Publishing)
If you live in the United Kingdom, go out to the White Cliffs of Dover and nearby areas and walk along the base of the cliffs. Weathering out of the soft chalky limestone (made entirely of coccoliths, as we just mentioned) are hundreds of small heart urchin shells from the Late Cretaceous. Originally, they were the subject of a classic study by Rowe (1899), who thought that they provided a clear case of gradual transformation from smaller shells with primitive characteristics (derived from the ancestral form Epiaster) to shells that are broader, with the tallest and broadest parts shifted forward. The groove in the front deepens and fills with tiny bumps, and the mouth shifts forward with a more prominent lip. The area for the tube feet lengthens and straightens out (fig. 8.9). However, later research showed that Rowe’s sampling was inadequate and he did no statistical studies at all. In 1954, Kenneth Kermack (since updated by Nichols [1959], Ernst [1970], and Stokes [1977]) performed a more rigorous analysis and found that, although the sequence was not a single smooth linear trend, there is still a remarkable branching sequence of species that partially overlap in time (fig. 8.9), from Micraster leskei to Micraster cortestudinarum (“tortoise heart”) to Micraster coranguinum (“eel heart”), and a side branch leading to Micraster corbovis (“cow heart”) and Micraster gibbus. These fossils are no longer evidence of gradualism within a single unbranched lineage, but there is still a good evolutionary sequence of transitional forms.
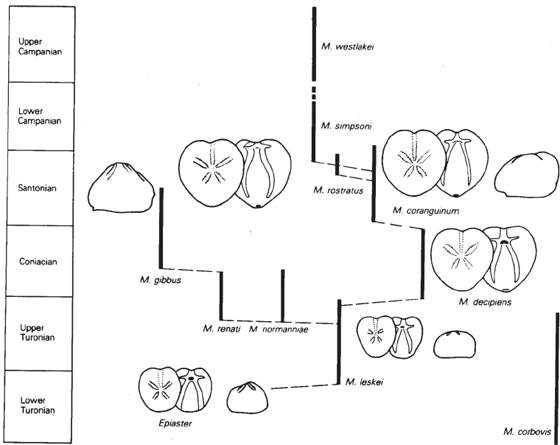
FIGURE 8.9. Evolutionary trends in the heart urchin Epiaster and Micraster from the Cretaceous chalk beds of the White Cliffs of Dover. (From Nichols 1959; courtesy of the Royal Society of London)
In the Jurassic beds of England to west of the White Cliffs, there are many additional excellent fossil sequences. A classic one of these is the weird oyster known as Gryphaea. Called “devil’s toenails” by the collectors, these creatures had one shell that was shaped like a coiled saucer that lay flat on the bottom (concave side up), and the other shell was much smaller and formed a lid on top (fig. 8.10). In a classic study, Trueman (1922) argued that Gryphaea gradually became more and more tightly coiled until the coils actually impeded the opening of the shell. In the thinking of the time, this was evolution run amok, developing to the point of obsolescence and no longer under the control of natural selection. But later, more careful studies by Philip (1962, 1967), Hallam (1968, 1982), Gould (1972), and Hallam and Gould (1974) showed that Trueman had misinterpreted his data. There is a trend toward less tightly curved and thinner, more dish-like shells that are much wider than long (fig. 8.10), but it happens in a number of different species lineages through the Jurassic, and much of the change in shape is primarily due to growth to a larger size.
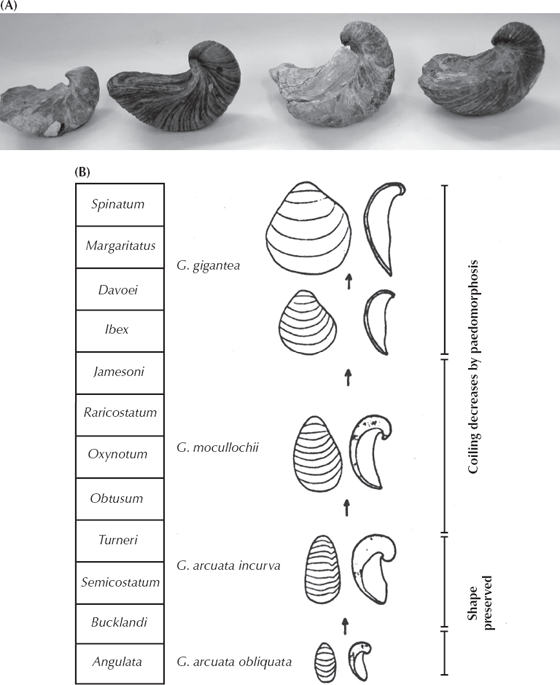
FIGURE 8.10. Evolution within the Jurassic oyster Gryphaea. (A) A series of Gryphaea shells, showing their decreasingly tight coiling through time. (Photo by the author) (B) The sequence of less tightly coiled shells found in the Jurassic beds of southern England. (After Hallam 1968: fig. 26; courtesy of the Royal Society of London)
Many other examples from the mollusks could be added to this list. For example (Rodda and Fisher 1964), the marine snail Athleta is very common in the Eocene (from 55 to 34 million years old) rocks along the Gulf Coast of Texas, Louisiana, Mississippi, and Alabama (fig. 8.11). They evolved from a simple nonornamented shell of Volutocorbis limopsis but quickly branched into several very different lineages. In the Wilcox beds, there is the huge, broad shelled Athleta tuomeyi, as well as the more normal lineage of Athleta petrosa. In the Weches beds, there are side branches to the nonornamented Athleta dalli and the more bulbous Athleta lisbonensis. The main Athleta lineage concludes in the upper Eocene Lisbon beds with the heavily ornamented Athleta petrosa symmetrica.
FIGURE 8.11. Evolution in the marine snail lineage Athleta from the Eocene beds of the Gulf Coastal Plain. (From Rodda and Fisher 1964; courtesy of the Society for the Study of Evolution)
Or how about everyone’s favorite fossils, the trilobites? Many different studies have been published on their patterns of evolution. In most cases, they show trends that are due to very subtle changes, such as the changing number of ribs in the thorax (fig. 8.12), as documented by Peter Sheldon (1987) on a 3-million-year-long sequence of over 15,000 specimens from eight trilobite lineages from the Ordovician of central Wales. Some lineages, such as Ogyginus, show almost no net change in rib number (stasis), while the nileids, Ogygiocarella, and especially Nobiliasaphus show a dramatic increase in rib number over the interval. This example shows both stasis and gradual evolution, but the conclusion is clear: these trilobites were not instantaneously created but keep changing through time. Other groups of trilobites show additional subtle changes through time, such as more complex eyes, increased size, more complicated tail segment (pygidium), and the development of spines (Eldredge 1977; Fortey and Owens 1990). Most of these examples are not gradual like those documented by Sheldon but show punctuation and stasis—and yet, contrary to creationists’ misconceptions, they do change through time, so they are good examples of evolution (just not gradual evolution).
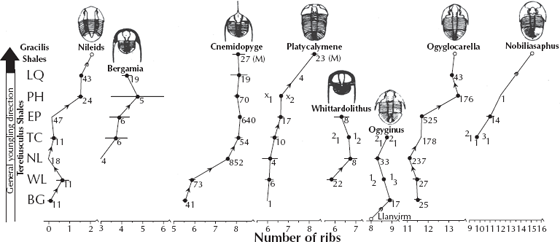
FIGURE 8.12. Evolutionary trends in numerous trilobite lineages from the Ordovician beds of central Wales. Most lineages show a gradual increase in the number of rib segments in the thorax through the different time intervals of the Ordovician. (After Sheldon 1987; copyright the Nature Publishing Group)
I could go on and on with many more examples such as these, but for space reasons, I will move on to the next topic. I recommend reading about some of the many examples documented in Hallam (1977), Boardman et al. (1987), McNamara (1990), Clarkson (1998), and Prothero (2013a) if you are interested in further documentation.
There is no need to apologize any longer for the poverty of the fossil record. In some ways it has become almost unmanageably rich, and discovery is out-pacing integration: the growing number of species of Foraminifera that remain undescribed in the cabinets of the oil companies probably is of the order of thousands; and while most other organic groups are not so fully collected the ratio of added finds to palaeontologists studying them is constantly expanding. But what remains to be discovered is likely to be of less and less radical importance in revealing major novelties, more and more of detailed infilling of fossil series whose outlines are known. The main phyla, in so far as they are represented by fossils, now have a long and full history that is made three-dimensional by a repeatedly cladal phylogeny. The gaps are being closed not only by major annectant forms, the “missing links” that Darwin so deplored…. Together, the discovery of new fossil forms, the filling out of the details of bioserial change, the interpretation of biofacies, the adoption of new techniques both in fossil morphology and in fossil manipulation, and the establishment of a progressively refined timescale contribute to a present-day palaeontology offering the strongest support, the demonstrative “proof,” of the fact and the process of evolution in terms wholly concordant with the essence of Darwinian theory.
—T. George Neville, “Fossils in Evolutionary Perspective,” 1960
When you point out the examples that we just discussed to the creationists, they weasel out of the problem by arguing that these changes are all within the “created kinds” as described in the Bible. As we pointed out in chapter 5, however, the concept of “kind” has absolutely no biological meaning, but creationists use it as a convenient dodge to allow changes on the microevolutionary scale (whatever evolves is within one kind) but to deny that major macroevolutionary changes could occur. Of course, this concedes that a heck of a lot of evolution is taking place, because nearly every group in the fossil record shows some evolution, and they can’t all be created kinds—and they couldn’t even all fit on Noah’s ark, as we already mentioned in chapter 3. But let’s play by their absurd rules and look at some radical changes in body form and ecology that are clearly macroevolutionary.
How about sand dollars? These cute little flat shells that are so popular with tourists and beachcombers are actually related to sea urchins and heart urchins and their kin. They are members of the phylum Echinodermata that also includes sea star, brittle stars, and sea cucumbers as well as many extinct groups. Living sand dollars are very flat and spend much of their time just below the sea bottom, buried with a light coating of sand. When they are feeding, however, they maneuver themselves with their short fuzzy spines and tube feet so that they stick out diagonally from the seafloor like a set of shingles (fig. 8.13A). Their mouth are on their undersides and face into the current, allowing them to trap food particles. Looking at a sand dollar, it seems nothing like any other “kind” on the seafloor, and it seems hard to imagine that it could evolve from something else—but it did! As documented by Porter Kier (1975, 1982), sand dollars evolved rapidly in the late Paleocene and early Eocene from the biscuit-shaped urchins known as cassiduloids to the slightly flatter oligopygoids to an even more flattened transitional fossil known as Togocyamus from the late Paleocene of Togo, West Africa (fig. 8.13B). In addition to getting flatter and flatter, sand dollars also show a progressive reduction in the size but an increase in the number of spines and bumps on the shell, so they go from rough and spiny to almost “furry” with tiny spines and bumps (which makes burrowing easier). The mouth, which had been on the leading edge of the bottom of the shell, shifts to the middle of the base of the shell, and the anus, which had been on the top side of the shell in primitive forms, shifts to the back edge of the bottom surface. Later in their evolution, they developed little holes and notches on the edge of the shell, which help modify the water flow around the shell as they lie buried. All of these transformations are well documented in specimens from the Paleocene and early Eocene (65–40 million years ago), starting in the central West African region, and eventually spreading worldwide.

FIGURE 8.13. The evolution of sand dollars. (A) Living sand dollars in feeding position, half-buried obliquely into the sand like shingles, with their mouths on the bottom facing into the food-bearing water currents. (Photo by the author) (B) Evolution of flat sand dollars from the biscuit-shaped cassiduloid sea urchins and slightly flatter oligopygoids through the transitional Paleocene fossil Togocyamus from western Africa and concluding with progressively flatter and more specialized sand dollars. The petal-shaped areas on the top of the shell bear the tube feet, while the mouth is on the bottom of the shell and gradually shifts from the center of the bottom to the lower front edge. Meanwhile, the anus shifts from the center of the top of the shell to the back edge of the shell as burrowing ability becomes more specialized and improved. (After Mooi 1990; used with permission of the Paleontological Society.)
Or how about a classic “living fossil,” the horseshoe “crab”? They are familiar to anyone who has combed the beaches of the Atlantic Coast of the United States, since they wash up frequently. During particularly high tides once a year, hundreds of them crawl up on the beach, mate, and lay their eggs in the sand in an orgy straight out of prehistory. Yet horseshoe “crabs” are not true crabs but instead are members of the group known as the Chelicerata that includes spiders and scorpions. (True crabs are a family within the Crustacea, a different group entirely.) Surely, they don’t look like any other group of arthropods, and if they are supposedly unchanged through millions of years, they would have no transitional forms that could produce the “horseshoe crab kind” from some other kind? Wrong! When I collected in the Upper Cambrian beds of Wisconsin, one of the most spectacular fossils I encountered were the plates of the primitive arthropods known as aglaspids (fig. 8.14A). These large creatures don’t look like the modern horseshoe crab, but still they are either close to their ancestry (Newell 1959; Fisher 1982, 1984) or related to both the horseshoe crabs and trilobites (Briggs et al. 1979; Briggs and Fortey 1989), so both trilobites and horseshoe crabs can be traced back to a fossil that looks like neither. Through the rest of the Paleozoic, there are additional species of the horseshoe crab lineage (subclass Xiphosura, “sword tail” in Greek), which develop progressively larger and larger head shields and fewer thoracic segments and, unique to the group, the long tail spine that gave them their name. Finally, by the Jurassic we find specimens of Mesolimulus walchi, which are very similar to the living species Limulus polyphemus, although their thorax is still not as heavily fused together and is much more spiny than the modern species. Thus xiphosurans haven’t changed much in the past 100 million years, but they changed a lot before then. Plus, there are weird experiments such as Austrolimulus fletcheri (fig. 8.14B), which has long boomerang-shaped spines on the corners of its head shield (appropriately, it is from the Triassic of Australia), or Liomesaspis (fig. 8.14C), which is reduced to two smooth button-shaped shields for the head and thorax and almost no tail spine.
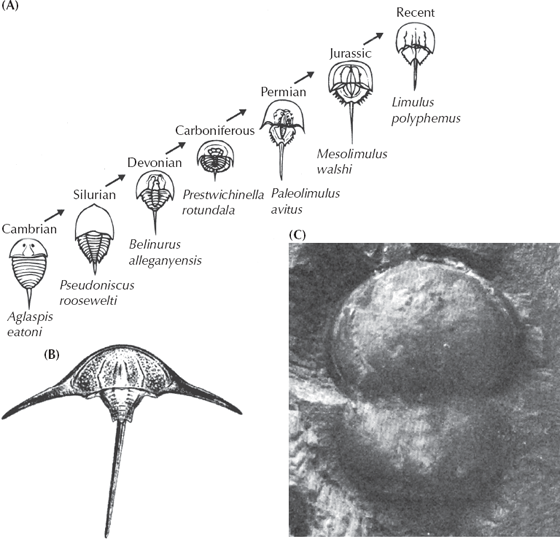
FIGURE 8.14. Evolution of the horseshoe crabs. (A) Evolutionary trends within the lineage from the primitive Cambrian aglaspids through more and more specialized and modern-looking horseshoe crabs. (B) The weird “boomerang-shaped” Australian horseshoe crab known as Austrolimulus. (C) The peculiar double-button-shaped horseshoe crab known as Liomesaspis. (Part (A) from Newell 1959; by permission of the American Philosophical Society; (B and C) courtesy D. Fisher)
Still not macroevolutionary enough? What about the “missing links” between major body plans and classes and phyla? Here, the problem is more difficult, because the great radiation of animals in the Cambrian was largely among phyla with soft, unfossilizable bodies before large shells finally appeared in the late Early Cambrian, or Atdabanian. We have excellent molecular, anatomical, and embryological evidence demonstrating how the major animal phyla are interrelated (fig. 5.7). This evidence shows that the mollusks are most closely related to the segmented worms among the living animal phyla. How about a transitional form between a worm and a mollusk? Ask and ye shall receive. Among the earliest fossil mollusks known from the Cambrian are simple cap-shaped shells that had been given names (such as Pilina), even though we had no evidence of their soft anatomy. Then, in 1952 a dredge brought up specimens from the deep waters off Costa Rica that included another classic “living fossil” named Neopilina galatheae (fig. 8.15). Neopilina is clearly a mollusk with a cap-shaped shell secreted by a mantle, as well as a mouth, digestive tract, anus, and gills.
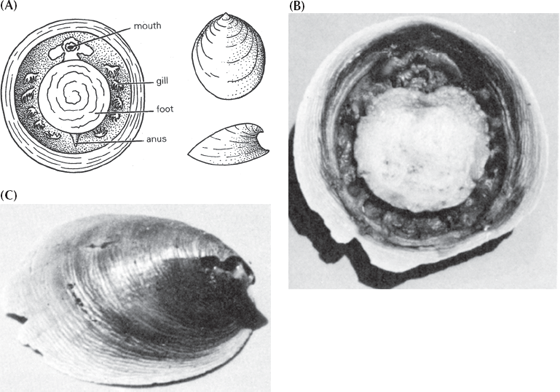
FIGURE 8.15. The “living fossil” Neopilina, a relict of the early Cambrian and a transitional form between segmented worms and mollusks. (A) Diagram showing the segmented paired gills on each side of the body (there are also segmented paired retractor muscles as well). This demonstrates that normally unsegmented mollusks evolved from segmented ancestors such as the annelid worms (confirmed by the molecular data). (B) Photograph of the underside of the living animal, showing the relict segmentation. (C) Top view of the cap-shaped shell. (Courtesy J. B. Burch, University of Michigan)
But it is unlike any other mollusk alive today because it still retains the segmentation of its wormlike ancestors. Arranged around the body on the margin of the mantle and below the lip of the shell are segmented gills, kidneys, hearts, gonads, and paired retractor muscles to pull down the shell. You couldn’t ask for a more classic transitional form. It is almost completely molluscan, yet it still has features of its segmented worm ancestry. Since 1952, scientists have come to realize that two soft-bodied wormlike groups, the Caudofoveata and the Aplacophora, are actually very primitive mollusks as well, even though they have no shells and look more like worms in their external form. We now have a nice transition from segmented worms to shell-less wormlike mollusks to mollusks with shells but with relicts of segmentation, and finally to the great radiation of unsegmented mollusks, including clams, snails, squids and octopuses, and all their extinct relatives.
How about transitional forms for the largest, most diverse phylum in the world, the Arthropoda, or “jointed legged” animals, which includes insects, spiders, scorpions, crustaceans (crabs, shrimp, lobsters, and barnacles), horseshoe crabs, trilobites, centipedes, millipedes, and many other groups? The tree of life (fig. 5.7) shows that arthropods are more closely related to nematodes (roundworms) and rotifers among living organisms. How could we imagine a transition between a nematode “kind” and an arthropod “kind” such as a millipede? It turns out that the transitional forms are still alive today; they are known as “velvet worms” or phylum Onychophora (fig. 8.16), pronounced “on-ih-KOFF-o-ra.” There are about 80 species of these creatures, living mostly in the tropical jungles of the world; they were originally mistaken for “slugs” when they were first described in 1826. But more careful observation (fig. 8.16) shows that although they may look superficially wormlike, they have many features of arthropods as well. Unlike the unsegmented nematode worms, onychophorans are segmented and have legs that resemble those of caterpillars. Their partially segmented legs end in horny hooked “claws.” Onychophora have cuticles made of the protein chitin, just like arthropods, and periodically have to molt in order to get larger (a feature found elsewhere only in the arthropods). They also have antennae, compound eyes, and mouthparts that are much like those found in arthropods. As outlined by Brusca and Brusca (1990:683), there are many other features that unite onychophorans with arthropods and make them outstanding transitional forms between the phylum Nematoda and the phylum Arthropoda.
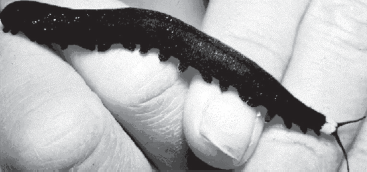
FIGURE 8.16. The living transitional form between worms and arthropods, known as the “velvet worms” or phylum Onychophora. Although they have segmented wormlike bodies, they also have jointed appendages and antennae and shed their cuticle like arthropods do. (Photo from IMSI Master Photo Collection)
The clincher is that we also have them in the Cambrian as well. The Burgess Shale in Canada and the Chengjiang Fauna of China produce amazing fossils of a variety of marine onychophorans known as lobopods, including Aysheaia (fig. 8.17A) and Hallucigenia (fig. 8.17B) from the Burgess Shale and Microdictyon from Chengjiang. Aysheaia is almost indistinguishable from some modern onychophorans, but Hallucigenia and some of the other Cambrian forms show an amazing array of spines and other features, giving the group much more diversity than we would appreciate from seeing only their living relatives.
FIGURE 8.17. Some examples of marine lobopod onychophoran fossils from the middle Cambrian Burgess Shale of Canada. (A) Aysheaia. (B) Hallucigenia. (Photos courtesy S. Conway Morris)
Once arthropods evolved, they diverged into a wide variety of body forms, from elongate multilegged centipedes and millipedes, to six-legged insects and eight-legged spiders, to the huge diversity of crustaceans. How did the onychophoran “kind” turn into the millipede “kind,” the insect “kind,” the spider “kind,” the crustacean “kind,” and so on? As we saw in chapter 4, the answer lies in the Hox genes and their ability to dramatically change body form through small changes in gene regulation. We have already seen how minor changes in the Hox genes produce homeotic mutants such as flies with legs on their heads or with four wings (fig. 4.5). Arthropods are particularly suited to this type of evolution because they have a modular construction with multiple segments, and each segment bears appendages that can be easily changed from a leg to a wing to an antenna to a pincer to mouthparts. Experiments have shown that a few Hox genes cause arthropods to add or subtract segments, and other Hox genes can produce whatever appendage is needed (fig. 8.18). Ronshaugen et al. (2002) put a shrimp Ubx Hox gene into an insect larva and showed how this gene was responsible for suppressing the development of limbs in insects (which have 6 legs, compared to the 10 in most crustaceans). Lewis et al. (2000) and Pearson et al. (2005) have shown how by manipulating Hox genes you can get just about any type or number of appendage on each segment of an arthropod, therefore making radical changes in body plan with a simple gene change. And there are many fossils that show primitive arthropods with more than two sets of wings, as in dragonflies, showing that the creature in figure 8.18 is not imaginary (Kulakova-Peck 1978; Raff 1998). Ironically, creationists attacked the first edition of this book by focusing just on one figure, figure 8.18, and claiming that it’s “made up.” Because of their ignorance, they didn’t realize there are fossils of insects with six, eight, and even more wings, so if anything, this multiwinged insect is a bit too conservative.
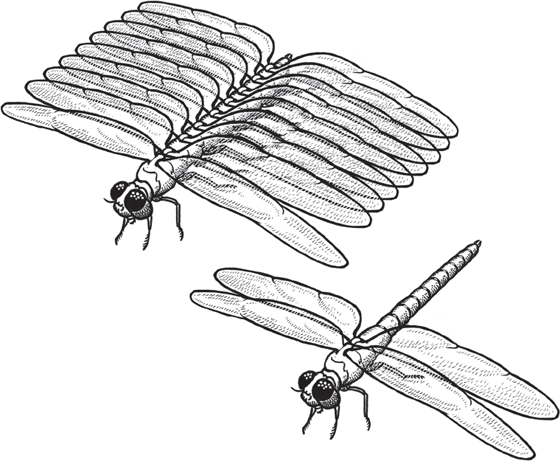
FIGURE 8.18. The evolutionary mechanism by which Hox genes allow arthropods to make drastic changes in their number and arrangements of segments and appendages, producing macroevolutionary changes with a few simple mutations (see fig. 4.6). (Drawing by Carl Buell)
In addition, arthropods can undergo radical changes in body form each time they shed their exoskeleton during molting. Think about how radically the body is rearranged from a caterpillar to an adult moth or butterfly, or from a maggot to an adult fly. Thus we can experimentally show that macroevolutionary transition from one body form to another with a completely different number of segments and appendages is a very easy process. No wonder arthropods are the most successful, abundant, and diverse organisms on earth. After we humans are long gone, the cockroaches and other insects will still rule the earth, as they have for over 300 million years so far.
In summary, the molecular-anatomical-embryological phylogeny of the animal kingdom (fig. 5.7) links mollusks and annelids; we have transitional forms, both fossil and living, between these two phyla. It also links nematodes and arthropods, and we have transitional forms, both fossil and living, between these two phyla as well. Using Hox genes, we can demonstrate how radical changes in body plans are controlled by relatively simple genetic mechanisms and allow macroevolutionary changes to take place. As we shall see in the next chapter, we also have abundant transitional forms that link the vertebrates and the echinoderms and show us the earliest ancestry of the vertebrates from soft-bodied ancestors.
Benton, M. J., and P. N. Pearson. 2001. Speciation in the fossil record. Trends in Ecology and Evolution 16:405–411.
Boardman, R. S., A. H. Cheetham, and A. J. Rowell, eds. 1987. Fossil Invertebrates. Cambridge, Mass.: Blackwell.
Clarkson, E. N. K. 1998. Invertebrate Palaeontology and Evolution. 4th ed. Oxford, U.K.: Blackwell Science.
Eldredge, N., and S. M. Stanley, eds. 1984. Living Fossils. New York: Springer-Verlag.
Fisher, D. C. 1982. Phylogenetic and macroevolutionary patterns within the Xiphosurida. Proceedings of the Third North American Paleontological Convention 1:175–180.
Gould, S. J. 1972. Allometric fallacies and the evolution of Gryphaea. Evolutionary Biology 6:91–119.
Hallam, A. 1968. Morphology, palaeoecology, and evolution of the genus Gryphaea in the British Lias. Philosophical Transactions of the Royal Society of London B 254:91–128.
Hallam, A., ed. 1977. Patterns of Evolution as Illustrated in the Fossil Record. New York: Elsevier.
Hallam, A. 1982. Patterns of speciation in Jurassic Gryphaea. Paleobiology 8:354–366.
Haq, B. U., and A. Boersma, eds. 1978. Introduction to Marine Micropaleontology. New York: Elsevier.
Kier, P. M. 1965. Evolutionary trends in Paleozoic echinoids. Journal of Paleontology 39:436–465.
Kier, P. M. 1975. Evolutionary trends and their functional significance in the post-Paleozoic echinoids. Paleontological Society Memoir 5:1–95.
Kier, P. M. 1982. Rapid evolution in echinoids. Palaeontology 25:1–10.
Kukalova-Peck, J. 1978. Origin and evolution of insect wings and their relation to metamorphosis, as documented by the fossil record. Journal of Morphology 156:53–125.
Lazarus, D. B. 1983. Speciation in pelagic Protista and its study in the microfossil record: a review. Paleobiology 9:327–340.
Lazarus, D. B. 1986. Tempo and mode of morphologic evolution near the origin of the radiolarian lineage Pterocanium prismatium. Paleobiology 12:175–189.
Lazarus, D., H. Hilbrecht, C. Spencer-Cervato, and H. Thierstein. 1995. Sympatric speciation and phyletic change in Globorotalia truncatulinoides. Paleobiology 21:975–978.
Lazarus, D. B., R. P. Scherer, and D. R. Prothero, 1985. Evolution of the radiolarian species-complex Pterocanium: a preliminary survey. Journal of Paleontology 59:183–221.
Malmgren, B. A., and W. A. Berggren. 1987. Evolutionary change in some late Neogene planktonic foraminifera lineages and their relationships to paleoceanographic change. Paleoceanography 2:445–456.
Malmgren, B. A., and J. P. Kennett. 1981. Phyletic gradualism in a Late Cenozoic planktonic foraminiferal lineage, DSDP Site 284, southwest Pacific. Paleobiology 7:230–240.
Malmgren, B. A., W. A. Berggren, and G. P. Lohmann. 1983. Evidence for punctuated gradualism in the Late Neogene Globorotalia tumida lineage of planktonic foraminifera. Paleobiology 9:377–389.
McNamara, K. J., ed. 1990. Evolutionary Trends. Tucson: University of Arizona Press.
Miyazaki, J. M., and M. F. Mickevich. 1982. Evolution of Chesapecten (Mollusca: Bivalvia, Miocene-Pliocene and the biogenetic law. Evolutionary Biology 15:369–409.
Pearson, P. N. 1993. A lineage phylogeny for the Paleogene planktonic foraminifera. Micropaleontology 39:193–232.
Pearson, P. N., N. J. Shackleton, and M. A. Hall. 1997. Stable isotopic evidence for the sympatric divergence of Globigerinoides trilobus and Orbulina universa (planktonic foraminifera). Journal of the Geological Society of London 154:295–302.
Prothero, D. R. 2013. Bringing Fossils to Life: An Introduction to Paleobiology. 3rd ed. New York: Columbia University Press.
Raff, Rudolf A. 1998. The Shape of Life: Genes, Development, and the Evolution of Animal Form. Chicago: University of Chicago Press.
Rodda, P. U., and W. L. Fisher. 1964. Evolutionary features of Athleta (Eocene, Gastropoda) from the Gulf Coastal Plain. Evolution 18:235–244.
Sheldon, P. R. 1987. Parallel gradualistic evolution of Ordovician trilobites. Nature 330:561–563.
Smith, A. B. 1984. Echinoid Palaeobiology. London: George Allen and Unwin.
Ward, L. W., and B. W. Blackwelder. 1975. Chesapecten, a new genus of Pectinidae (Mollusca: Bivalvia) from the Miocene and Pliocene of eastern North America. U.S. Geological Survey Professional Paper 861.